The fossil-based economy is represented today by crude-oil refineries obtaining fuels for transport to be used as feed for combustion engines (e.g., gasoline, jet fuel), high value chemicals and petrochemical raw materials. All refineries have been constructed after different motivations and design strategies. The refineries appeared in the 1940’s using just a low percentage of the accessible crude-oil and throwing away (polluting with) very complex residues that today are fully considered for high added value products. Actually, in the refineries about 85% by mass of refined crude-oil corresponds to fuels and the remaining percentage (15%) consists of other products [1].
However, all the crude-oil refining was developed historically step by step adding routes and products depending on the market and needs defined by the society through the history without considering environmental impacts, as for example Greenhouse Gas (GHG) emissions [2]. All the non-valorized fractions were considered residues to be disposed in landfills or open ponds. It means that no design strategies for integral valorization of the crude-oil were proposed at the beginning. Today, and after a big pressure from the society based on the environmental issues as well as the energy and materials demand, all fractions of crude-oil are practically used to obtain different products as it is discussed for example in the Indian refineries case [3]. In the world there are two types of refineries, state and private and all refineries use technologies highly predictable and optimized, namely mature technologies [4]. A classical definition of petroleum refineries according to the North American Association of Industry Classification Systems (NAICS) [5], consider it as installations primarily engaged in processing crude petroleum into different fractions. This definition is also followed by the U.S. Energy Information Administration in official documents 2015 [6]. Current refineries include a deep and integral analysis based on the thermodynamics, phase equilibria, mass balances as well as energy consumption in different units as preliminary crude-oil fractionation, cracking (thermal and catalytic), hydrotreating, reforming, hydrogen generation and others related to catalytic and separation processes.
Actually, there are different modern approaches for designing and operation of refineries using one or different types of crude oil. Some strategies are based on the scheduling optimization when crude oil types are mixed [7]. A modular refineries approach including simple diesel production units or more advanced cracking refineries are considered flexible and cost-effective for crude producers on small scale as it was demonstrated in the case of Nigeria [8]. One of the most comprehensive handbooks including these and other approaches as well as the most significant and recent changes to petroleum refining was launched on 2018 by Coker [9]. The book additionally includes modelling, calculations and simulation using the software UniSim. It is important to note that the most important developments reached last years in sustainability for oil refineries are related to computers and programming advances, making the consideration of a huge number of variables during the design stages possible. For that, optimization strategies to get the best configuration are usually used, including the Model-Based Mixed-Integer Programming Approach commonly used in process engineering [10].
The biorefineries can be considered as evolution of the biofuels concept in the world. A biorefinery should be defined as a complex facility (or network) that involves integral biomass conversion processes to produce a range of products, mainly biofuels, power, materials, food and feed as well as chemicals and biochemicals based on biomass [11]. A biorefinery can be seen as a homologous process to a refinery in which a raw material of natural origin is divided into different products [12]. For this, diverse bibliography related to the use of organic waste to obtain value-added products can be found [13]. Usually biorefineries use different types of biomass or organic wastes after processing biomass such as glycerol (obtained after biodiesel production from oils) to obtain high added-value products [14]. As many concerns can appear when biomass can be used for food or bioenergy, the use of second and third generation raw materials is preferred in biorefineries to avoid land competition [15].
The Task 42 [16], considers the existence of two types of biorefineries depending on the final use of the products. The energy-driven biorefineries involve those cases, where the final purpose is energy production. The main products in this case are biofuels, power and/or heat [17]: biofuels (biogas, syngas, hydrogen, biomethane, pellets, lignin for combustion, charcoal, biodiesel, bioethanol, FT-fuels, bio-oil) and electricity and heat [16]. The product-driven biorefineries consider as a main purpose to obtain products that are not used for energy generation:
Chemicals (fine chemicals, building blocks);
Organic acids;
Polymers and resins;
Biomaterials;
Food and animal feed;
Fertilizers.
Some products in this type of classification can be used as energy products and platform chemical products [18]. For example, bioethanol and biohydrogen can be used in both classifications. Then, finally the specific product classification will depend on the final use of the product in the context of market demand [17].
Recently, a most modern definition of Biorefinery was introduced: “A biorefinery is a complex system, where biomass is integrally processed or fractionated to obtain more than one product including bioenergy, biofuels, chemicals and high value-added compounds that only can be extracted from bio-based sources. The latter after a comprehensive study of the raw materials to be used and a sustainable design based on the latest state of the art technologies and approaches which include aspects of the three pillars of sustainability” [19].
When looking for sustainability concept in biorefineries many strategies for right designs are discussed in the literature. The definition of sustainability should be included not only as a goal but also as a rule for designing based on the most classic definition of sustainability according to the Brundtland report “sustainable development is development that meets the needs of the present without compromising the ability of future generations to meet their own needs” [20]. It is considered that sustainability encompasses in an integral and equitable way three concepts to be included and validated through robust calculations: economy, environment and social aspects [21]. In this sense, different authors have proposed many design methods in order to integrate this concept into the design of biomass-based processes. Cardona et al. [22] proposed the knowledge-based approach, which focuses on the conceptual design of biorefineries as well as on the technical, economic, environmental and preliminary social analysis. Jenkins et al. [23] propose the life cycle assessment as a useful tool to determine the environmental impact of biorefineries as essential part of the sustainability characterization of the process. Demirel et al. [24] also discuss this approach for Lignin Biorefineries. Espinoza et al. [25] have involved the study of supply chains in the analysis of sustainability for biorefineries considering uncertainties and strategic, tactical and operational criteria. A more practical definition and use of sustainability concepts is proposed through the delimitation of the design spaces of biorefineries by considering stakeholders’ concepts and information. Authors applied this concept for designing a jet biofuel production process in Brazil [26]. The proposed strategy can be a good way to solve the problem of weights for economic, environmental and social components of the sustainability concept, that usually are not objectively defined. On the other hand, Parada et al. [27] proposes a practical analysis to incorporate the sustainability in the design of biorefineries that incorporates the analysis of the supply chain for the biorefinery, which is according to the circular economy concept. It is important to note that logistics is part of the design and it is totally connected with the main principles of circular economy: to preserve and enhance natural resources, to optimize resource yields and to foster system efficiency (biorefineries preserve resources and reduce pollution when integral use of biomass is considered in the design. This statement is possible only when biomass is available to be used in the proposed process or biorefinery) [28].
In this work, different multiproduct biorefineries at different levels of scale, maturity or application were analysed and discussed as it is shown in Table 1.
Biorefineries described in the present work as cases of study based on biomass availability and nature (every case will be described later in sections 2.1 and 2.2, but for detailed description readers can refer to the original references)
Raw material | Status | Comments |
---|---|---|
High scale ethanol and polyhydroxybutyrate production based on coffee residues. | Proposed | Restricted by logistics. Non standardized feedstock. |
Medium scale ethanol and furfural production based on Pinus Patula residues. | Proposed | Low logistics restriction. Standardized feedstock. |
Low scale ethanol, xylitol and antioxidants production from blackberry residues. | Proposed | Logistics restriction. Non standardized feedstock. Low scale possibilities. |
Energy-driven biorefinery for producing biodiesel and ethanol based on palm. | Proposed | Non logistics restriction. Standardized feedstock. High scale possibilities. |
Low scale production for oil, pulp, antioxidants and flours based on amazonian fruit. | Proposed | Logistics restriction. Non standardized feedstock. Low scale possibilities. |
Medium scale biorefinery for bioproducts from oil palm residues. | Proposed | Non logistics restriction. Standardized feedstock. |
High scale production of sugar, ethanol, energy, anthocyanins, polyhydroxybutyrate from sugar cane. | Proposed | Non logistics restriction. Standardized feedstock. |
Biorefineries and plants implemented at industrial level. | In operation | Non logistics restriction. Standardized feedstock. |
It is important to note, that usually the concept of biorefinery is so misunderstood that the quantity of real cases (in operation) should be considered as dramatically low. Most of the biorefineries in the practice are stand-alone facilities that in the last years migrated to a convenient concept of biorefinery without any integral and well-designed process for the maximum use of biomass to obtain a range of different products (multi-product portfolio). So, in this paper the last case, reported in Table 1, groups 4 plants in operation (or recently were in operation) together with two types of thermochemical conversion of biomass in a biorefinery way. Additionally, the proposed cases are deeply assessed by the original authors and it includes a sensitivity analysis in terms of feedstocks composition and scale (please refer to the bibliography in sections 2.1 and 2.2). Every biorefinery is described and discussed briefly based on the literature and previous works. For this, the products and design strategy is discussed and compared to refinery analogies. As it is covered in Table 1, the status, type of feedstock, logistics, scale and the range of products make these biorefineries different and interesting for any analysis. Moreover, through these examples (Table 1) the main challenges to boost the biorefineries in the near future can be discussed.
From Figures 1 and 2, the analogy between refineries and biorefineries can be understood. However, at the same time the differences are very clear. In crude-oil refineries the location, compositions, technologies and products are practically defined and the uncertainties are minimal. However, for the biorefineries, even if the purpose is the same, the uncertainties could be very high and these aspects are very relevant. Additionally, the location and the scale are the key factor for the sustainable operation of the facility and the products should be defined after long heuristics and conceptual design through analysis of alternatives.
Refinery design based on mature technologies, products and market
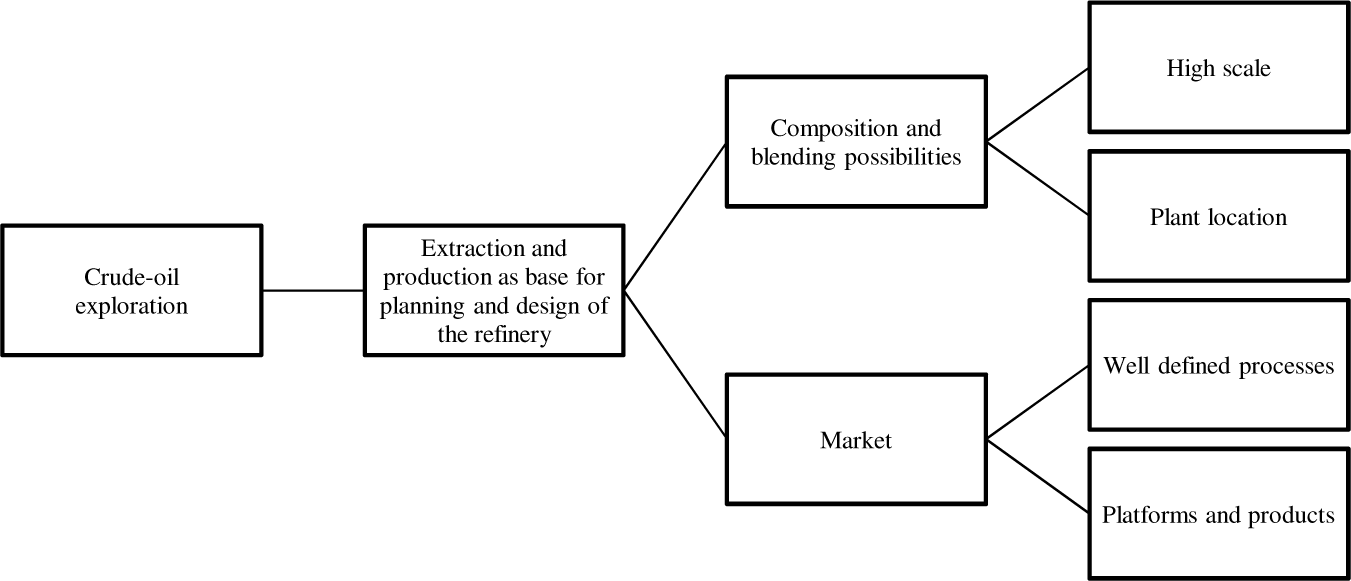
Biorefinery design based on biomass availability and nature
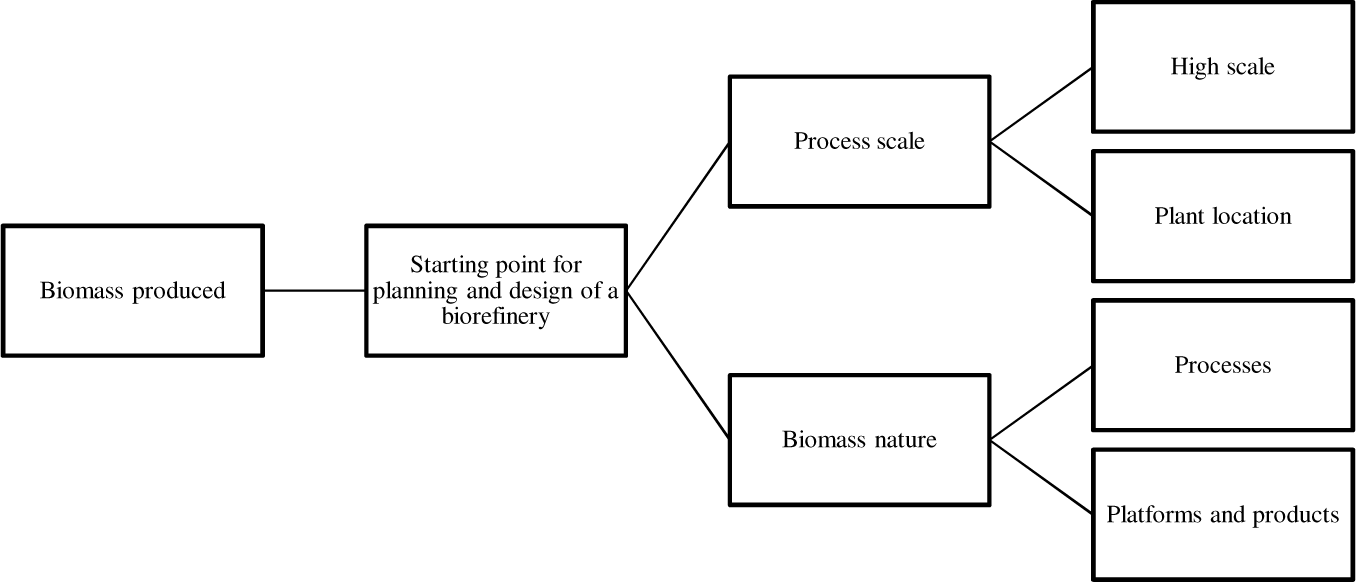
The hydrocarbons mixture (i.e., crude-oil) and the biomass as raw materials will define exactly the most important differences during the design according to the homogeneity and maturity in technologies. The qualities of crude-oil are different due to the type of soil from which it is extracted. This leads to obtain a crude-oil with different physical and chemical properties. The biomass characteristics depend on supply management as for example on time of maturity and exposure to air, sun, humidity, etc. Additionally, the presence of oxygen is very limited in crude-oil and abundant in biomass. Most of the products obtained at high scale from hydrocarbons can be summarized in fuels as natural gas, liquefied petroleum gases for transport and energy, polyesters and nylon for textiles, polyurethane for adhesives and boards, polymers for bags and packing, glycols for cosmetics, ammonia for fertilizers, synthetic rubbers for tyres, asphalts for roads and insecticides for human health and agriculture among hundreds of other products. The main purpose of the biorefineries is to replace these products with the biomass-based molecules [22]. However, currently the demand for petrochemicals continues as a result of the consumption and energy increase associated with the population growth mainly in developing countries. The lifestyle also changes dramatically in developing countries presuming an aggregate rate of increase in energy and petrochemicals consumption in the world. One issue with the projected growth in demand for petrochemicals is the feedstock and product prices depending on policies and sometimes geostrategic policies and wars. The market as a global and complex system strongly affects the biorefineries business. Usually when the crude-oil is expensive in the market, biorefinery products or biofuels are interesting and the project financing dramatically increases, disappearing finally when any global factor returns the price to lower values. To develop and extend the real access to alternative feedstocks to many countries, biomass prices stabilization as well as maturity in technologies are needed.
Additionally, the carbon footprint for fossil fuel usage involves carbon dioxide (CO2) emissions well considered and punished by the society. It is considered that the climate change benefits derived from biomass utilization is based on the balance given by the CO2 absorbed during the growth of that biomass [26]. However, in recent years this point can be criticized and every case will need specific demonstrations.
The market evolution for petrochemicals defined the sustainability of the hydrocarbons industry. In the beginning just some percentage of the crude-oil was used and a huge quantity of residues polluting the environment appeared. However, at that time no concerns about the environment and the increased demand for energy (as a result of the progress) made possible this type of non-sustainable industry. As the technologies for fractionation and conversion were developed together with a demand for new products, as for example plastics, the crude-oil as raw material began to be more and more integrally used and the residues reduced to the low levels that the industry knows today [9]. Moreover, at that time the quality and efficiency of the informatics was so low that it is really difficult to compare to the software and other tools available today. For biomass as raw material, this approach cannot be applied. The boom of biorefineries should be analysed in a new sustainability context that today is considered as mandatory. Even if biomass is a complex system and the maturity on the technologies are not so high, it is necessary to involve sustainability principles and strategies as discussed in Table 2 during exhaustive design.
Overall sustainability principles and design strategies to be applied in biorefineries in comparison to the crude-oil refineries based on design strategies for sustainable biorefineries [19]
Refinery design strategy | Biorefinery design strategy | Comments |
---|---|---|
Refining a same quality of crude oils and in some cases specific blends. However, in many cases, associated feedstocks as natural gas were not fully considered. | Integration of feedstocks. As high as integration can be applied, the overall biomass efficiency increases as well as the raw material utilization. | The biorefinery strategy should continue. New refineries now incorporate the use of other feedstocks within the plant as natural and shale gas LEXINNOVA. |
Integrated technologies were the best strategy applied in last decades in petrochemicals production as for example reactive distillation. | Integrated technologies as fermentation together with saccharification or separation in one unit is the most efficient. | Biorefineries adopted integrated technologies from the petrochemical industry. |
The reduction of waste streams was not a key objective in refineries. With time it changed and design strategies as pinch analysis were involved. | Multiprocessing biorefineries based on the different levels of integration: different raw materials and products combination to obtain other added value products. | Biorefinery design included from the beginning approaches from crude-oil industry as for example the pinch analysis. |
Refineries design began with standalone facilities without a conceptual analysis of the possible and convenient products to be obtained. | The multiproduct portfolio is always the best case. As the number of products increases the process indexes (technical, economic, environmental, social) usually increases [29], [30]. | Any project based on biomass should consider, based on preliminary or heuristic analysis, the maximal number of products to be obtained after full utilization of biomass. |
The refineries for many years didn’t care about the environment and generally the ecosystems. Today the crude-oil industry image is not so good mainly due to this well known. | Second and third generation raw materials preserves ecosystems, reducing the use of other natural sources. | Biorefineries should not repeat these errors and the environmental impacts analysis must have the same importance as the economic assessment adopting the concept of Life Cycle Analysis (LCA). |
Crude-oil industry has the most developed and efficient logistics implementation. However, the real social impact is very low. Just for example, in terms of distribution oil is a privilege for some countries. | Biomass is the most distributed raw material in the world existing in all the countries. If the logistics is working properly together with an efficient biorefinery all the impacts could be positive. | The logistics and socioeconomic aspects of the biorefineries are more important than in oil refineries. The supply chain will define the real success of the project considering the huge quantity of biomass that can be distributed along the countries or regions. |
In crude-oil refineries for many years energy consumption and very complex byproducts and residues were not an issue. Energy was considered as very abundant and residual fractions of oil or byproducts were just disposed in landfills or open ponds. | By-products valorization and reduction in energy consumption is mandatory during design. | Energy consumption and byproducts utilization or valorization are also an essential part of the biorefineries design to decide the viability if the project. |
Crude-oil industry was the engine for thermodynamics, kinetics, mass-energy transfer and other topics development. From the beginning this industry designed and developed the most powerful design software. | Biorefineries assessment in terms of sustainability is an essential part of the design. The use of Aspen Plus and other software for specific units is a rigorous way to analyse the convenient scale of the processes [31], [32]. | Biorefineries design practically uses all the design software from crude-oil industry. An specific software is needed to be more close to the real feedstocks and technologies used in biomass conversion. |
Even at early stages, crude-oil refineries were very concerned about safety based on state of art methodologies for robust calculations. | Design safer processes. The use of chemicals and materials under controllable conditions is a goal of the good simulation to ensure stability of the units. | Biorefinery design uses the same strategies form crude-oil industry to consider the safety. |
Crude-oil industry and petrochemical plants practically never considered past, current and future scenario for oil refining (including allocation factors, market products, etc.). However, it is well explained by the stable market we have today for fuels and polymers for example. | To understand the biorefinery possibilities sensitivity analysis could be included in the design [33]. | Scenario and optimization strategies can provide information about the real sustainability of the biorefinery before it is implemented. New crude-oil refineries need also ungently this type of analysis based on the real risk that biomass represents for them. |
The crude-oil business has established a quota production models to regularize prices. Even at different uncertainties this model is still working. | As proposed [34], the hedging strategies should be considered to avoid unexpected and not desirable changes in prices for raw materials and energy used in the biorefinery. This makes possible to manage financial risks. | An organization as Organization of countries exporting crude-oil (OPEC) could be needed to stabilize the business model in terms of biomass prices. |
If scientists, industry and governments try to accomplish the biorefinery concept as a basis for future bioeconomies, not repeating the errors learned from crude-oil industry at the beginning, some challenges should be solved:
Biomass as raw material should be considered integrally. The design of biorefineries could analyse the alternatives of fractionation and valorisation of all the biomass compounds. For example, the use of biomass for obtaining in standalone way (considered anyway by some authors as biorefineries against the definition discussed before) high added value antioxidants but leaving up to 99% in mass of residues is not possible, not welcome and totally contradictory;
The products to be obtained should combine the market, the real possibilities and the context in every case. In this case, even the more aggressive standalone processes as the thermochemical processing of biomass should address the concept of biorefinery.
Process design aims to transform certain material through physical and/or chemical stages into a new material with different features than the original material and with higher benefit [17]. The processing is decomposed into a number of units or blocks for intermediate transformations through different steps of reaction, separation, mixing, heating, cooling, pressure change, and particle size reduction or enlargement, among others [35]. Process design is affected by multiple elements. The first element is the operation units, which undertake the unitary processes/operations for a specific goal (reaction, purification, physical transformation, pressure change, and heat exchange, among others). The second element is the streams that interconnect the units. The third and fourth elements focus on the excellent use and exploitation of energy and mass within the process. In other terms, processes should use raw materials as efficiently as is economic and practicable, and same for energy consumption [35]. Finally, the previous elements constitute the design scheme, which is the process scheme and final element of the process. Figure 3 summarizes the previously mentioned elements. All these elements apply in both crude-oil based (refineries) and biomass-based (biorefineries) processes. In terms of process design, different approaches and studies have been done for each one of these processes. The following sections will show the studiesand advances done in this regard.
Elements related to a process and its design
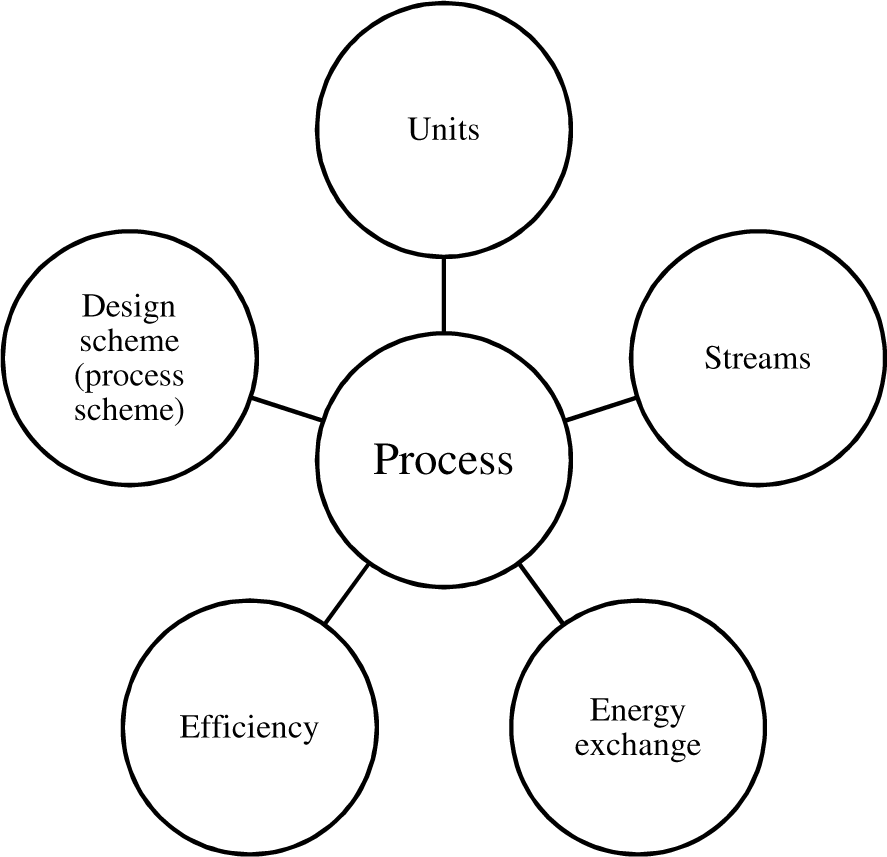
Chemical processes date from several centuries ago. These are mature and have established methodologies for their design and evaluation. Process design may have many solutions depending on the design goals. According to Mody and Strong [36], the goals of process design must be to eliminate non-optimal solutions with as little effort as possible. One of the most common group of methodologies combines the conceptual design and the hierarchical decomposition, to determine the step that most affects each process and to use this critical step as the base point for the design of the rest of it [20]. Due to this, the methodologies for designing biotechnological processes had to be reconsidered in terms of specific analysis of processing stages that differ considerably from chemical processes [35]. This type of hierarchy is generally represented through an “onion” diagram, as it is shown in Figure 4.
“Onion” diagram for the hierarchical design of process [35]
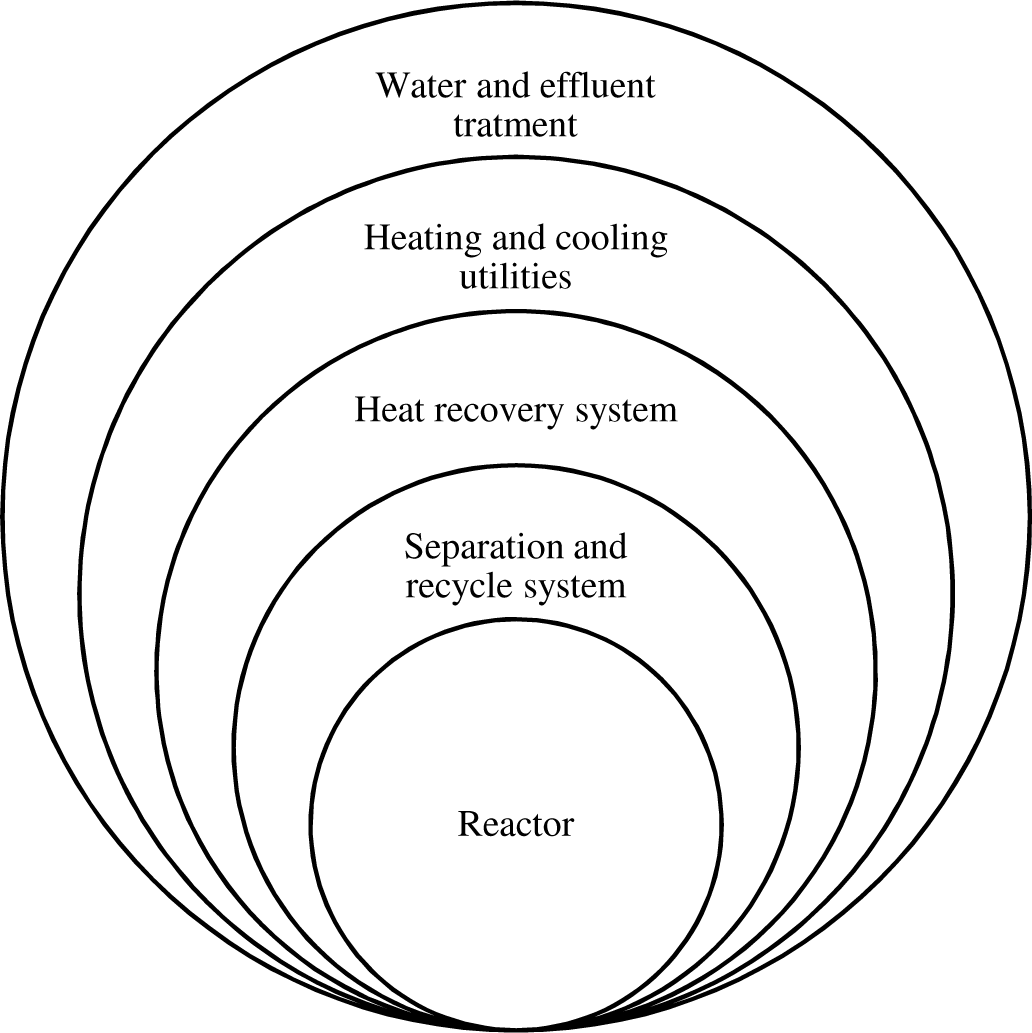
Officially as the United Nations states, biotechnology is any technological application that uses biological systems, living organisms or derivatives thereof, to make products or processes for specific uses [37]. These processes open a new spectrum of possible raw materials, which were currently considered as mere residues (e.g., biomass). During the last decade, biotechnological processes aimed at a wide variety of processes. Several products (biofuels, bioenergy, food products, biomaterials, biomolecules and chemical products) have been developed [38] and different raw materials have been explored, mainly coming from agroindustrial processes. Due to this, the methodologies for designing biotechnological processes had to be reconsidered in terms of specific analysis of processing stages that differ considerably from chemical processes [39]. In this regard, the most advanced concept up to date is the biorefinery, producing different products such as biomolecules, biomaterials, bioenergy and biofuels through an efficient technological scheme [40]. The design of biorefineries and further considerations will be described as follows.
For the design of biorefineries, multiple models have been proposed. Moncada et al. [38] proposes the design and evaluation of biorefineries from the knowledge-based approach. Kelloway et al. [41] propose a guided approach to biorefineries optimization. Pham et al. [42] discuss this approach as an improvement of the process synthesis. Kokossis et al. [43] use the same strategy but considering biomass conversion systems as the basis of any analysis. Patel et al. [44] propose an Early-stage approaches approach. In the design of a biorefinery, it is necessary to consider technical, social, and political aspects as well as logistics and laws, among others. The most common methods of design described by the mentioned authors are studied hereinafter:
Optimization. This design method aims to maximize the yield or the economic potential based on the limitations, chosen technology, conversions, reactants and products [27]. First, it is necessary to define the desired goal to achieve, for example, maximum production yield or minimum production cost [45]. Then, it is necessary to perform an analysis of the technology alternatives (conversion routes), defining the critical steps in the biorefinery, to integrate technologies and establish the bases of the economic analysis [46];
Early-stage approaches. This design method focuses on a preliminary assessment without requiring detailed process modelling. Then, the results are compared with the petrochemical equivalent process [47]. Therefore, it is necessary to perform a detailed assessment of the reaction system assuming a 100% recovery of the products [24];
Knowledge-based approach. This design method applies three concepts: hierarchy, sequencing and integration. These elements will be described as follows:
Hierarchy. The first level in a biorefinery is raw materials. A given raw material will allow obtaining certain type of products. Depending on the components of the raw material, it is only possible to obtain certain chemical building blocks that can be converted in specific products. Moncada proposed five families of products that can be obtained from biomass: biofuels, bioenergy (e.g., power and heat), food products, biomaterials and biomolecules [19]. Figure 5 shows the linkage between the types of biomass, obtained chemical building block and possible products. The second level of a biorefinery is the technologies. Hierarchy in this regard focuses on the step that most affects each process. This is a modification of the onion diagram [36], which considers the critical stage of the process in the center [19]. Depending on the desired platform, the critical stage may vary in a lignocellulosic-based biorefinery;
Distribution of biomass types, building blocks (platforms) and possible products
Sequencing. This stage aims to establish a logical order and relation between technologies and products [29]. Therefore, the first step must be the selection of desired products, definition of a main product and by-products and the available technologies. It is very important to define clearly the purpose of the biorefinery. To exemplify, from the production process of sugarcane, it is possible to obtain sugar and molasses as by-product. If the aim of the biorefinery is the production of ethanol, both products could be used for this end. However, if the aim is to produce both sugar for human consumption and ethanol, only molasses should be directed for the production of the alcohol [22];
Integration. This stage aims to take advantage of all the mass and energy streams within the process. Therefore, in this stage mass and energy balances become transcendental. Mass integration can refer to the optimization of the use of reactants and their recycle, extractive processes of recirculation, pervaporation processes for the separation of different components [48], for example. Roffler et al. [49], studied the extractive fermentation of acetone and butanol. Energy integration focuses on the design of a heat exchange network or the implementation of power cycles in order to improve the efficiency of each stage into the biorefinery [50];
Sensitivity analysis. This stage aims to identify the trends and tendencies of the biorefinery in terms of its economic and environmental performance in terms of a given input. This information is very important because it gives information about the scale and the feasibility of the process. This stage varies the percentage of the given input (e.g., feedstock flow) in a positive and negative margin and determines the margins of economic and environmental feasibility of the process.
Manufactured chemicals generally are classified in three groups according to the production volume. Bulk or commodity chemicals are produced in very large quantities and tend to have a low sales price. Fine chemicals are obtained in small amount and the price is higher. Specialty chemicals have the highest price per gram and their production volume is the lowest. Generally, commodity chemicals are produced through continuous operation mode and these processes are characterized by large plants, low profit margins, and high-energy consumption [51]. For specialty and fine chemicals, the most common operation mode is batch. These chemicals provide higher profit margins and have less cyclicality in their business cycle, generally, they are produced in volumes lower than 1,000 tons per year [38].
Necessarily, high production volumes will require high amounts of feedstock. However, the scale is not only related to the amount of generated product, but to the amount of processed raw material (processing scale). It is important to note that from the latter perspective, it is possible to obtain both bulk and specialty chemicals in small scale and large scale of production. Nonetheless, the benefits associated to a large scale of production would not be the same as in the case of a large scale of feedstock processing.
According to production capacity, biorefineries can be classified into small and large scale. The size of a biorefinery is influenced by important factors: government and environmental policies and market conditions [52]. These elements influence directly on supply chain decisions, technology selection and integration levels. To exemplify both types, a small-scale biorefinery is the extraction of essential oil, colorants and pectin from fruit peel, a large-scale biorefinery is the production of biodiesel, ethanol and electricity from palm. This definition of scale includes both perspectives (raw material and product), because it depends on the amount of feedstock and hence this limits the production of a given product.
The small or large scale concept is something very confused when biorefineries are designed and specific references or rules are needed. For this purpose, it is necessary to look for another reference that considers certain types of processes or stages of a process with respect to the processing/production scale, which will be addressed in the following section. The aim of this section would be to identify some ranges of raw material flow or capacity that define the scale of the process.
Pilot plants are systems developed after the commercial opening of a given process, with the main purpose of gathering information about operating conditions, productivities and so on. Typically, pilot plants are smaller than full-scale production plants, and some of them can be built based on lab equipment. In other cases, considerable engineering and financial efforts are necessary. Therefore, the differences of pilot scale and demonstration scale are strongly influenced by the industry and the application of the obtained product. According to Hellsmark et al. [53], in the pharmaceutical industry, pilot scales tests are performed with loads of 20-100 kg, and in the petroleum industry bench scale systems are with less than 1 L of catalyst, pilot plants will have a catalyst volume between 1 and 100 liters, and demonstration plants have catalyst volumes in the 100-1,000 L range.
According to the International Energy Agency (IEA) Bioenergy Task 42: Biorefining [54], currently there are some biorefineries already settled and working and it defines the “state-of-the-art” of the plant and the capacity the plant handles. Table 3 shows some of the plants described in the report. It can be observed that the increasing order of capacities for each type of plant is pilot plant, demonstration plant and commercial scale, but there is no clear range of processing flow of raw material from which goes any of these scales. In general terms, pilot plants process raw material in orders of magnitude of 1-10 ton/day, demonstration plants go in orders of magnitude of 10-100 ton/day and commercial scales start from 100 ton/day or higher. However, there are some commercial scale plants that define their processing capacity within the range of pilot plants. Other authors report that small-scales and pilot plant range can go around 2-25 ton/day or 1,500-7,500 ton/year of input of biomass and that at commercial level, small-scale is within the range of 100-500 ton/day of input of biomass for bioenergy production, and 5,000-15,000 ton/year of product for biochemicals.
Examples of different “state-of-the-art” plants considered actually as biorefineries [54]
Name | Location | State-of-the-art | Feedstocks | Outputs | Capacity |
---|---|---|---|---|---|
Bomaderry Plant | Australia | Commercial scale | Wheat and wheat flour | Wheat gluten, wheat starch, glucose, ethanol, stock feed | Production of 300 × 106 L p.a. |
BDI bioCRACK Pilot Plant | Austria | Pilot plant | Lignocellulosic biomass (wood chips, straw) | Raw diesel fuel, pyrolysis-oil, char | Input capacity: 2.4 ton/day |
AGRANA Biorefinery Pischelsdorf | Austria | Commercial scale | Agrarian raw materials | Bioethanol, wheat starch and gluten, CO2 | Wheat starch facility: processing 250,000 ton/year (684 ton/day)Bioethanol production: processing 500,000 ton/year (1,370 ton/day) to produce 210,000 m3/year of ethanol (165,700 ton/year-454 ton/day) |
Alberta Pacific Forest Industries | Canada | Commercial scale | Wood | Kraft pulp, bio-methanol, heat and power | Processing capacity: 765,000 ton/year (2,000 ton/day) |
Maabjerg Energy (Project) | Denmark | Commercial scale | Wood chips, straw, manure, sewage sludge and Municipal Solid Waste (MSW) | Bio-methane, bioethanol, fertilizer, electricity and heat | Processing capacity: 300,000 tons of straw, 520,000 tons of manure, 280,000 tons of various types of bio-waste, and 100,000 tons of MSW per yearProducts: 80,000 m3 of bioethanol, 50 × 106 m3 biogas, 36,000 tons lignin |
INBICON | Denmark | Demonstration plant | Straw, corn stover, bagasse and Empty Fruit Bunch (EFB) | Bioethanol, electricity, heat and feed | Production: 5.4 × 106 L/year of ethanol (4,260 ton/year-12 ton/day) |
CELLULAC | Ireland | Commercial scale | Lactose whey permeate and lignocellulosic biomass | Chemicals [Lactic Acid (LA), Ethyl Lactate and Polylactic Acid (PLA)] | Capacity: 100,000 ton/year (280 ton/day) |
Beta Renewables – Crescentino | Italy | Demonstration plant | Giant reed (Arundo Donax), miscanthus, switch grass, agricultural waste (straws) | Bioethanol and animal feed | Capacity: 60,000 ton/year (170 ton/day) |
ICM | USA | Pilot plant | Corn fiber, switch-grass, energy sorghum | Fuel-grade ethanol and co-products (feed) | Processing capacity: 10 ton/day |
ZeaChem | USA | Commercial scale | Cellulosic feedstock (poplar trees, corn stover, wheat straw) | Bioethanol, ethyl acetate ester | Processing capacity: 10 ton/day |
However, these ranges cannot be ensured or assumed to be applicable for all the cases, types of products and feedstocks, as it can be seen from the previous examples. Each type of process, product and feedstock can have different ranges that can be considered as small, medium or large scale. This situation shows that there is no clear definition of scale and its ranges and even the considerations can be ambiguous.
To give a clear example of the ambiguity in the definition of small or large scale for a process, let’s consider a process to obtain a given metabolite of the peel of a fruit. Given the amount of the product of interest in the peel, the production volume will be low because it can be considered as a fine or specialty chemical and the scale in this could be considered as a small scale. However, once again given the low concentration of the product in the peel, it is necessary to process huge amounts of raw material in order to obtain the desired amount of product. In that case, the scale of the process with respect to the raw material would be higher. Therefore, in this regard two persons would have arguments to justify the process with two different scales. The main problem is that the term “scale” is used indistinctly to refer for the amount of raw material or product. Based on this, it is necessary to give clear definitions of both “categories” of scale, which is done in the following section.
A general definition of the scale of a process can be the capacity to process the raw material in order to obtain the desired product. It is common to find that the definitions for the scale of a process are generally associated to the production volume or the processing volume of raw material. There is a direct relation between the production volume and the feedstock requirement. However, the scale may not be related only to the product flow, but to the flow of processed raw material (processing scale). The common point between both approaches is that large scale is a high flow, whether it is from processed raw material or obtained product. The same applies for small scale. Considering this, three definitions of scale will be proposed as follows.
According to the supply of raw material, the definition of large and small scale is strongly associated to the availability of a given raw material. As it was previously mentioned, large scale in this case refers to high amounts of raw material that can be supplied to the process per unit of time. In this sense, the scale could be assimilated to the ‘processing scale’, which refers to the ‘amount of raw material processed in a period’. Therefore, a biorefinery that processes raw materials as sugarcane, palm, corn and their respective wastes could be considered as large scale, because the amount of feedstock that is available is very high.
According to the flow of products, the definition of large and small scale is strongly associated to the type of product that is obtained. Large scale in this case refers to high amounts of product that can be obtained in the process per unit of time. In this sense, the scale could be assimilated to the ‘production scale’, which refers to the ‘amount of product obtained in a period’. Therefore, a biorefinery that produces bulk chemicals as ethanol, sugar, butanol, biodiesel could be considered as large scale and one that produces lactic acid, Polyhydroxybutyrate (PHB), citric acid could be considered as small scale.
In small scale and large production scales, it is possible to obtain both bulk and specialty chemicals. Nonetheless, the benefits associated with a large scale of production would not be the same as a high processing scale. Generally, the design of chemical processes is not restrained by the amount of available feedstock, especially for the production of bulk chemicals. However, for the processing scale, this is a limiting factor directly related to the amount of obtained product.
Therefore, biorefineries can operate at small and large scales. An example of a small-scale biorefinery is using fruit peels for the extraction of essential oils, colorants and pectin. An example of a large-scale biorefinery is using palm to produce biodiesel, ethanol and electricity. In this case, both biorefineries include both processing and production scale perspectives (raw material and product). They depend on the amount of feedstock, the obtained product and the possible products are specialty/fine chemicals. This is why feedstocks as sugarcane and palm are used in large-scale biorefineries to obtain bulk chemicals as biodiesel, ethanol and electricity, because there is a high amount of feedstock available for their use. On the other hand, fruit peels and feedstocks in lower amounts are generally used for the production of specialty chemicals that have higher benefit.
The third definition of scale can be related to an economic approach. In this regard, it is necessary to perform an economic evaluation of the process and a sensitivity analysis of how the economic performance of the process [Net Present Value (NPV)] changes with the flow of raw material. Once the equilibrium point is identified (NPV at the end of the lifetime of the process equals to zero), this would be the start point to determine a “feasible small scale”. Then, in this case, small scale would refer to the ‘minimum processing scale at which the process presents a feasible economic performance’. This is a new term that will be considered as the Minimum Processing Scale for Economic Feasibility (MPSEF).
Based on the fact that it is possible to obtain both bulk and specialty chemicals in both small and large-scale biorefineries, it is necessary to recognize the most significant factors affecting each scale. According to several biorefineries (small and large-scale) designed with multiple raw materials (castorbean [30], residual banana [55], olive stone [56], Pinus Patula [33], glycerol [57], among others) and for the production of multiple products (energy [33], ethanol [30], butanol, PHB [55], antioxidants [57], among others), it is possible to observe two remarked differences associated with the scale. In both cases, generally the most representative costs are utilities and raw materials. For large-scale biorefineries, the cost of raw materials tends to be much higher than that of utilities.
This behave or is very common in industry and it can be explained by how the scale defines the impact of the size and productivity of the equipment on the Capex and Opex of any project. Generally, the cost per unit of output decreases with increasing scale (fixed costs are divided into more units of output) [37]. In this case, for small-scale biorefineries, the cost of the raw materials, logistics, transportation, procurement (despite of being residues in most cases), equals the cost of utilities because these last elements (cooling, heating and instrumentation air) must be fully supplied and it is not possible to generate significant integration networks. Hence, this means that utilities are more expensive in small-scale processes. Large-scale biorefineries require higher amounts of feedstock and proportionally the cost will increase, however, utilities can be further used through heat exchange networks that will decrease the cost of the utilities.
In this section, some cases of study for biorefineries will be discussed that have been evaluated from a technical, economic and environmental perspective using various raw materials. For each case, a description of the evaluated scenarios, the most relevant results and an analysis, taking into account the criteria described in the previous section, will be performed. The second part of this section includes the description of biorefineries already implemented on industrial level and also plants in operation for the production of energy and ethanol from a wide variety of raw materials.
In coffee production different residues such as pulp, husk, leaves, and coffee grounds are produced mainly during the coffee harvesting, processing and final consumption [58]. Other residues that are obtained in high volumes are coffee leaves and Coffee Cut-Stems (CCS). These last are obtained when coffee trees are cut to obtain a younger tree with higher coffee productivity. These wastes are seasonally produced and stockpiled for their burning or, in most of the cases, for their auto-degradation.
In García et al. [59], three possible scenarios for the processing of CCS are analysed. From the diagram shown in Figure 6, 3 scenarios were proposed. Scenario 1 is the production of hydrogen, scenario 2 the production of hydrogen and electricity and scenario 3 the production of hydrogen, electricity and ethanol. The configuration with the best economic and energy performance was the multiproduct portfolio (scenario 3). The most relevant results of this study are shown in the Table 4.
Biorefinery flowsheet based on coffee cut stems
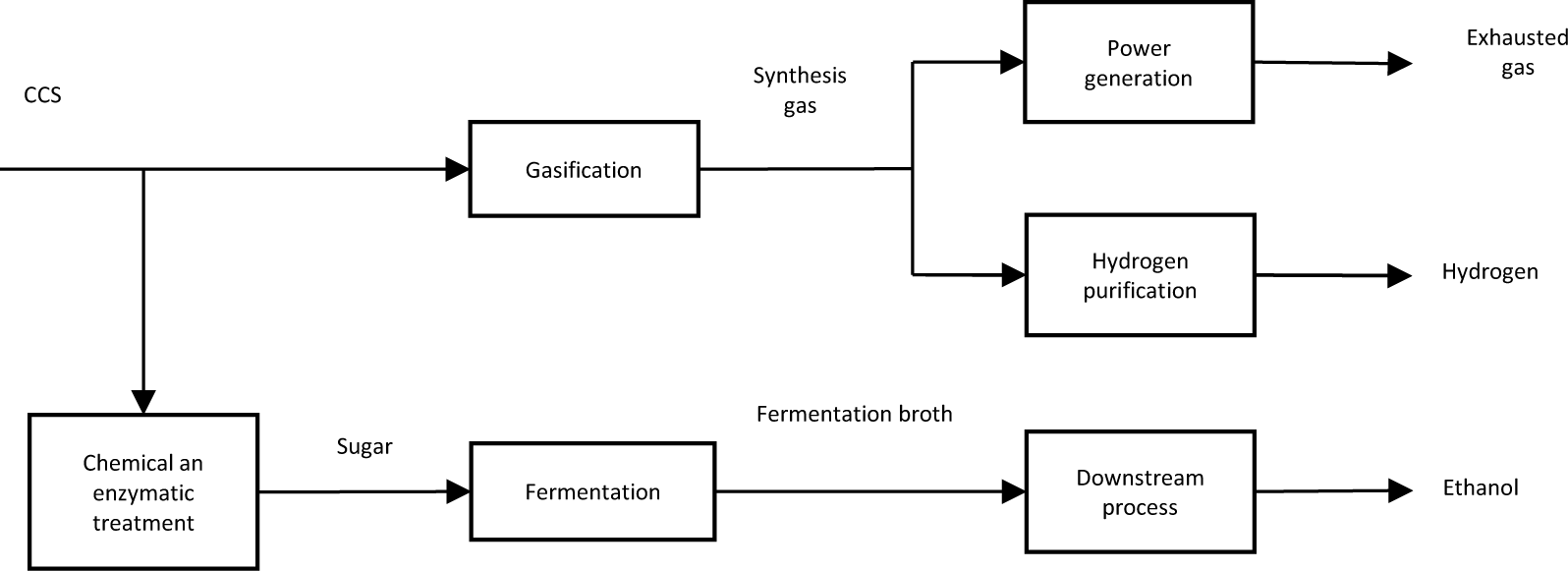
The most relevant results for a CCS biorefinery based on García et al. [59]
Scenarios | Productivity | Yield | Energy efficiency [%] | ||
---|---|---|---|---|---|
Value | Units | Value | Units | ||
Scenario 1 | 2.1 | [ton H2/day] | 0.042 | [ton H2/ton CCS] | 62.06 |
Scenario 2 | 0.72 | [ton H2/day] | 0.014 | [ton H2/ton CCS] | 58.2 |
2.94 | [MW] | 5,021.7 | [MJ/ton CCS] | ||
Scenario 3 | 0.68 | [ton H2/day] | 0.012 | [ton H2/ton CCS] | 94.5 |
10,244.1 | [L ethanol/day] | 202.2 | [L ethanol/ton CCS] | ||
1.2 | [MW] | 2,041.8 | [MJ/ton CCS] |
In this case most of the residues were valorized. However, an integral analysis was not applied. The logistics analysis developed at coffee industry level (confidential results and access) demonstrated later that the implementation of this project is practically impossible for Colombian context based on high inclination fields and the existence or more than 100,000 small farmers that dramatically increases the transport costs and the raw material price, even if it is a residue. Here the crude-oil refinery strategies cannot be used to improve these results. However, additional work is needed to demonstrate the lower scale possible to have a feasible project including the logistics restriction. It is important to note that this is mainly a simulation work and no experiments supported the results.
Pinus Patula is a soft wood grown widely and used in industry for producing important amounts of residues with just a small portion for specific purposes (for example, the sawdust is used in cement industry). The idea of a second-generation biorefinery based on lignocellulosic feedstocks (i.e., Pinus Patula bark) leads to the formulation and evaluation of biorefining alternatives to obtain different and new products.
Wood residues as Pinus Patula bark are an alternative feedstock for producing ethanol and furfural in Colombia as it was demonstrated through assessments developed in Moncada et al. [33]. Different pretreatment alternatives were tested (i.e., dilute acid, alkali, liquid hot water) as well as the fermentation of cellulose hydrolysate to produce ethanol (using S. Cerevisiae), and the dehydration of hemicellulose hydrolysate to obtain furfural. The chemical composition of Pinus Patula and the yields obtained from the experimental assessment were used to feed a biorefinery simulation (see Figure 7) using Aspen Plus to carry out the techno-economic and environmental assessment. Three scenarios were assessed and compared based on different levels of energy integration.
Biorefinery design based on Pinus Patula bark
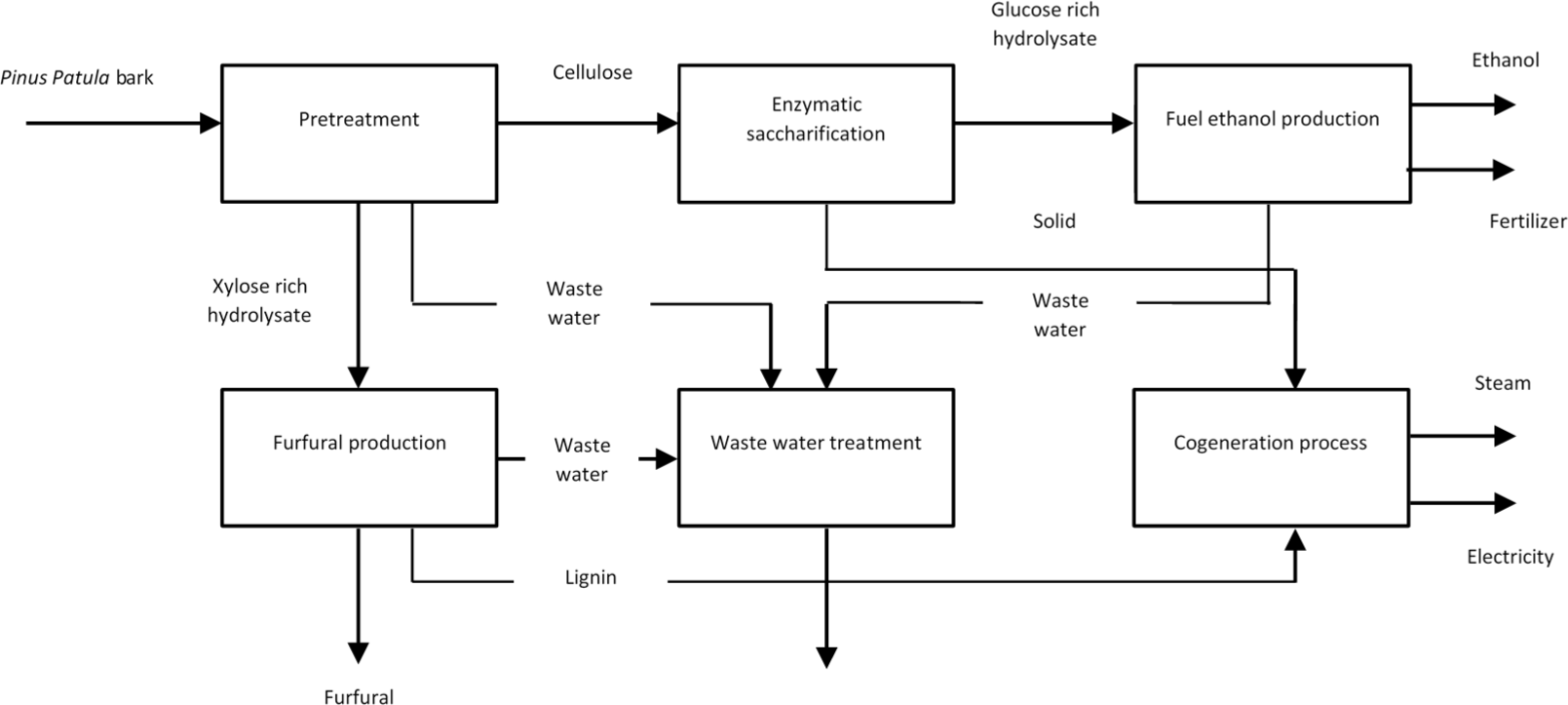
The alkali method showed the highest yield (50%). The pentose rich hydrolysate was used as substrate to produce furfural using sulphuric acid as catalyst (yield 91%). The cellulose saccharification was done enzymatically (max. concentration 63 g/L), and the hexose hydrolysate was used as substrate to produce ethanol using S. Cerevisiae as strain (0.42 g ethanol/g sugars). For the three scenarios based on different levels of heat integration (i.e., non-integration, full energy integration, full energy integration plus cogeneration). The fully integrated scenario plus cogeneration system was the configuration with the best economic and environmental performance. For this case, the economic margin was 37.96%, and the potential environmental impact was 0.063 PEI/kg products. This result served as basis to draw recommendations on the technological, economic and environmental feasibility to implement such type of biorefinery in Colombia. Table 5 shows the most representative results in the simulation of the biorefinery described.
Mass balance of all biorefinery scenarios
Raw materials | |
Pinus Patula bark [kg/h] | 40,000 |
Sodium hydroxide [kg/h] | 8,115.7 |
Sulfuric acid [kg/h] | 4,000 |
Enzyme [kg/h] | 2,000 |
Water [kg/h] | 442,000 |
Residues | |
Sodium sulfate [kg/h] | 5,636.68 |
Carbon dioxide [kg/h] | 3,896.34 |
Cogeneration system | |
LP-steam [kg/h] | 180,000 |
Gases [kg/h] | 176,323.37 |
Ash [kg/h] | 1,348.67 |
Products | |
Ethanol [kg/h] | 4,080.79 |
Furfural [kg/h] | 6,485.28 |
Fertilizer [kg/h] | 9,945.64 |
Water [kg/h] | 448,390.66 |
This biorefinery was designed through experiments at laboratory level and simulations with last tools for process design and analysis. The logistics in this case is not a restriction but some questions appear: is it really the best combination of products? Is there a real and stable market for the so called fertilizers and animal feed? It is important to note that the crude-oil refineries for example have a very defined and well-studied market to decide what kind of products will be involved in the plant. It looks today that most of the biorefineries proposed at scientific world are very weak in terms of market justification. It could be very dangerous because the errors at this stage can mean a future non sustainable business.
Andes Berry (Rubus glaucus benth) is a common fruit in many countries, especially in Colombia and other tropical countries. This fruit has an important composition in phenolic compounds, specially anthocyanins using an enhanced-fluidity liquid extraction process with CO2 and ethanol [60]. In 2011, Colombia had a production of 94,000 tons of Andes Berry fruits [61], which is used to produce concentrates, jams and juices among other products. However, the fruit processing leaves significant amounts of residues, which can be used in the extraction of anthocyanins with chemical, cosmetic, food and pharmaceutical purposes.
Spent Blackberry Pulp (SBP) is a waste product of blackberry juice extraction with a high potential as a raw material for valuable compounds. In Dávila et al. [62] a biorefinery is proposed for producing phenolic compounds extract, ethanol and xylitol from SBP (Figure 8). Techno-economic and environmental assessments were developed in order to analyse both, total production costs and potential environmental impact of the proposed biorefinery. Four potential scenarios were evaluated:
A biorefinery without mass and heat integrations as well as without a cogeneration system;
A biorefinery with heat integration but with neither mass integration nor cogeneration system;
A biorefinery with mass and heat integrations but without cogeneration system;
A biorefinery with mass and energy integrations as well as with cogeneration system.
Table 6 shows the results obtained in scenario 1.
Biorefinery design based on SBP
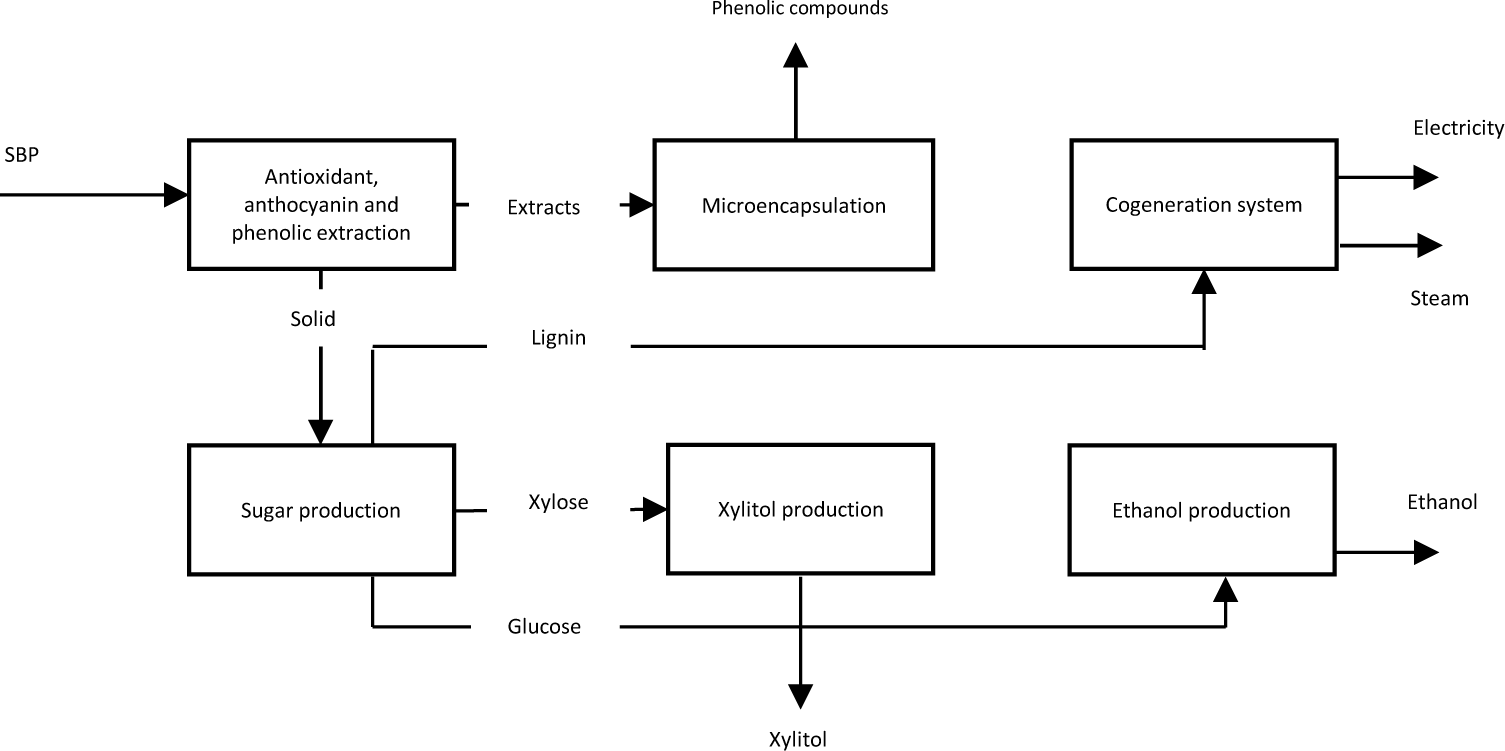
Results indicated that the integration significantly affected the total production cost obtaining a reduction of 16.1%, 32.97% and 30.90% for scenarios 1, 2 and 3 while scenario 4 (with cogeneration system) increases the total production cost of the biorefinery. The sale to total production cost ratio was calculated, finding that the scenarios with mass and heat integration with very high values (close to 25) become the most promising for obtaining value-added products. The environmental assessment reveals that potential environmental impact can be reduced according to the level of integration in the biorefinery.
Mass balance for the scenario 1 [11]
Raw material | ||
SBP | 2,000 kg/h | |
Products | ||
Product | Flow [kg/day] | Yield [kg/ton SBP] |
Xylitol | 452.2 | 18.37 |
Ethanol | 6,912 | 352.9 |
Phenolic compounds | 193.4 | 3.88 |
The crude-oil refineries in the world are mainly high scale facilities. Just in the beginning some small scale companies existed. The biorefineries can be high scale, but the small scale cases are not so common given that energy is usually the main purpose. The only solution to the scale problem is the added value. It is possible to affirm that the crude-oil refineries products are low added value and in the biorefineries case together with biofuels some high value chemicals can be produced. In the SBP biorefinery analysed here, the low scale is compensated with very high profits (sale to production costs ratio higher than 25). These values can be achieved in practice when molecules like antioxidants or pharmaceutical products can be obtained. Very high added values in crude-oil industry is something impossible today, but the market for any added value product is also very limited.
In Rincón et al. [63], two biorefineries based on oil palm were designed, assessed and compared to transform this feedstock into biodiesel, alcohols and other added value products. The first scenario considers the simultaneous production of biodiesel and ethanol from lignocellulosic biomass and glycerol. The second scenario includes palm oil fractionation, biodiesel production and biomass fired cogeneration using a gasification technology. Figure 9 presents the flowsheet for the two biorefineries. These two integration alternatives were analysed according to their potential income (total sales/total production cost ratio), and environmental impact (WAR algorithm). Table 7 shows the most relevant results obtained.
Oil palm biorefinery
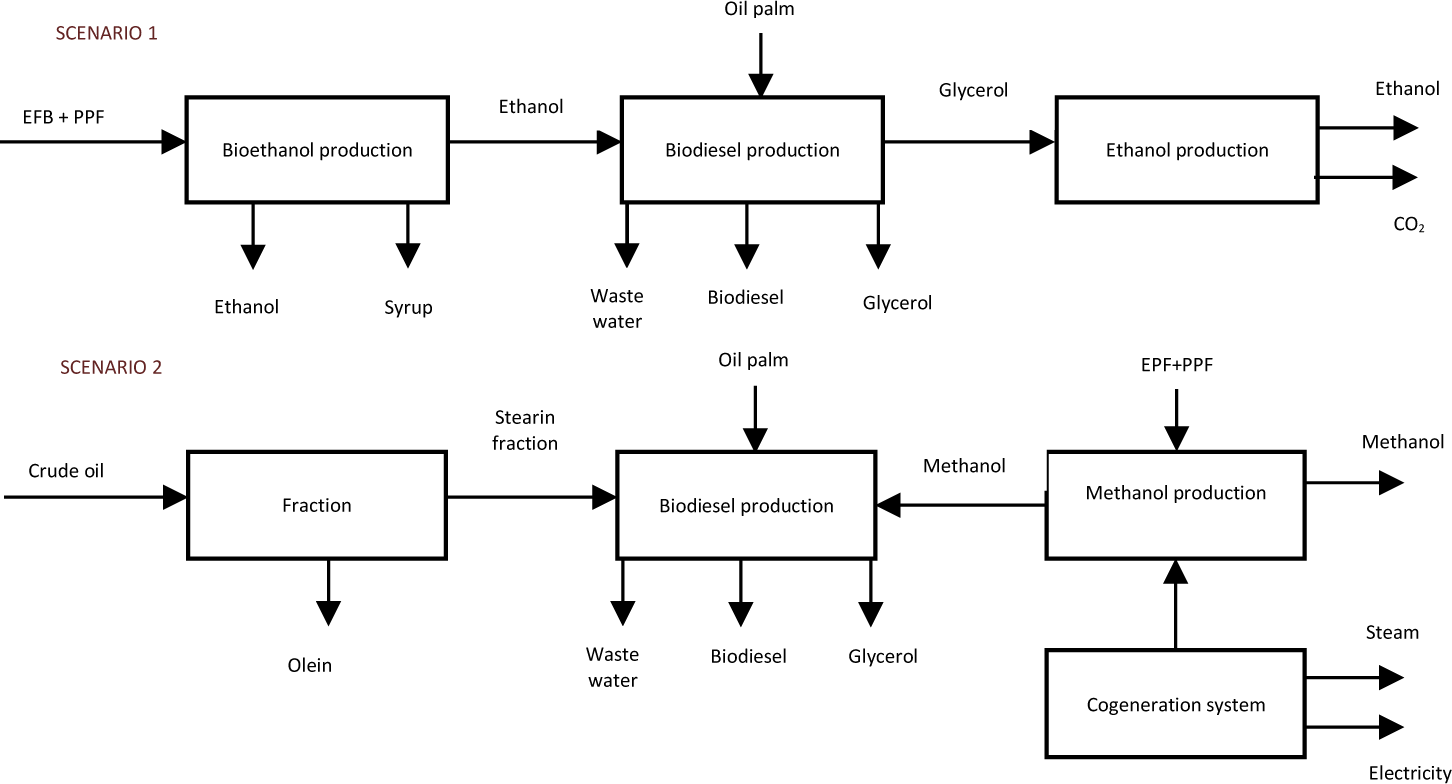
Mass balance of the two scenarios reported in Rincón et al. [63]
Raw materials | |||
The first scenario | The second scenario | ||
Crude palm oil [kg/h] | 2,916 | Palm oil [kg/h] | 37.37 |
Ethanol [kg/h] | 1,509.44 | Empty fruit bunches and palm press fibre [kg/h] | 246.71 |
Sodium hydroxide [kg/h] | 43.74 | Air [kg/h] | 2,000 |
Water [kg/h] | 80,662 | Sodium hydroxide [kg/h] | 35.63 |
Sulphuric acid [kg/h] | 503.01 | Process water [kg/h] | 720.61 |
Empty fruit bunches and palm press fibre [kg/h] | 18,828 | Sulphuric acid [kg/h] | 4,671 |
Ammonium [kg/h] | 12.341 | ||
Calcium hydroxide [kg/h] | 97.39 | ||
Glycerol [kg/h] | 323.01 | ||
Residues | |||
Waste water [kg/h] | 92,896 | Waste water [kg/h] | 5,509 |
Sodium sulphate [kg/h] | 61.78 | Flue gas [kg/h] | 2,177.62 |
Lignin [kg/h] | 1,848.68 | Sodium sulphate [kg/h] | 58 |
Gypsum [kg/h] | 1,526.35 | Ash, char and tar [kg/h] | 2,211.29 |
Energy | |||
No production | Electricity (generated) [MW] | 171 | |
Energy [MW] | - | ||
Heating | - | ||
Cooling [MW] | 215 | ||
Products | |||
Biodiesel [kg/h] | 3,041.55 | Biodiesel [kg/h] | 5,490 |
Glycerol [kg/h] | 294.49 | Glycerol USP [kg/h] | 616 |
Ethanol [kg/h] | 3,461.91 | Olein [kg/h] | 31.973 |
Syrup [kg/h] | 2,077.73 | Methanol [kg/h] | 46.872 |
Carbon dioxide [kg/h] | 96.61 |
The total sales/total production cost ratio obtained for the integrated approaches were 1.88 and 3.33, respectively. The economic and environmental assessment revealed a better global performance for the second integrated approach. In this sense, a biorefinery with a major number of products and low energy consumption is an important option for the development of oil palm industry. The latter is due to the maximum utilization this feedstock.
Palm is an interesting example of total integration of feedstocks in the framework of bioenergy production. The question about the use of methanol (mainly produced in crude-oil refineries) or ethanol (possible to be obtained from the lignocellulosic residues produced in the oil extractions units) demonstrated the competition between crude-oil and biomass industry. Additionally, the logistic problems for energy production in rural areas are also solved through this approach if considering that ethanol, biodiesel and electricity can be produced without dependency on supplies in raw materials from crude-oil refineries.
The actual Amazon Region is more than any natural region with high biological and ethnic biodiversity, of fragile ecosystems. It is an essential supplier into the global climate change and source of riches without exploitation. It is a large Cuenca that is shared for seven south-American countries. But, first and foremost, it is the scenario of the most serious social, environmental and territorial conflicts derived to the people movements without organization, resources exploitation and extraction, and public programs and mistaken regulation or lack of them. Last statements discussed in detail in Correa et al. [64] involves the justification for new proposals for boosting the economy in this region. In a biorefinery based on an exotic fruit as Makambo at low scales to produce: pasteurized pulp, seed butter, residual cake (it is a paste that should be used as an ingredient in a food industry) as a substitute for cacao, phenolic compounds, biogas and biofertilizer. This fruit belongs to Sterculiaceae family as Theobroma cacao (Cocoa) and Theobroma grandiflorum (Copoazú). It grows in different regions of Central and South America. Pulp is used for direct consumption as food. Seeds are used as a kind of cocoa. Peel is usually a waste. In Figure 10, the biorefinery is disclosed. Table 8 shows the material balance for the biorefinery described.
Amazonian fruit biorefinery: Makambo case
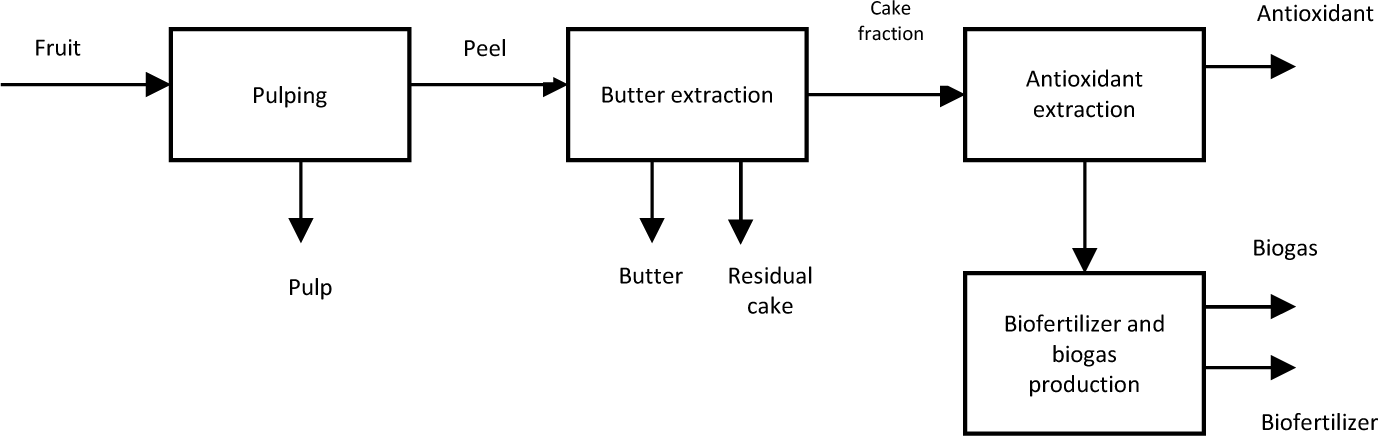
Mass balance for the oil palm biorefineries as reported in González et al. [65]
Raw materials | |
Makambo fruit [kg/day] | 1,000 |
Ethanol [kg/day] | 1.69 |
Carbon dioxide [kg/day] | 40.20 |
Water [kg/day] | 231.52 |
Residues | |
Waste water [kg/day] | 619.44 |
Carbon dioxide [kg/day] | 39.87 |
Products | |
Pasteurized pulp [kg/day] | 257 |
Residual cake [kg/day] | 56.54 |
Butter [kg/day] | 61.11 |
Phenolic compounds [kg/day] | 5.36 |
Biogas [kg/day] | 142.35 |
Biofertilizer [kg/day] | 91.35 |
As a result of the analysis, a positive NPV was found with a payout period of 5.1 years and a profitability index of 1.35. For this small scale it is a very representative result. On the other hand, the environmental analysis carried out with the WAR software resulted indicator total the contamination of 3E-2 PEI/kg product, in which the category that contributes most to this value is acidification potential.
The case analysed can be considered as very low scale project (2-5 tonnes a day as maximum). The solutions for small scale projects should integrate natural not very well processed products (with no sophisticated technologies) and very high added value products as antioxidants (with very sophisticated technologies). The crude-oil refineries at fractionation steps also use very simple technologies as distillation with plates and only to obtain very high special chemicals complex catalytic reactors and sophisticated separation units are used. But the most important result of this work, where experimentally and theoretically the feasibility of this biorefinery was demonstrated, is the possible use of a biorefinery concept at small scale to solve problems in small rural communities.
Oil palm is grown in Colombia in four different zones, which are southwestern, northern, central and eastern. The oil palm crop became one of the most important oil crops in the world due to the following features: steady and continuous oil production, adsorption of large amounts of CO2, low biodiversity compared to the crops that it replaces. In addition, its production yields are higher than those achieved by other oil crops such as soybean, rapeseed and sunflower. Oil Palm Rachis (OPR) is a residue coming from the oil palm tree. Oil palm fronds are the largest lignocellulosic biomass that is produced during the oil palm tree harvesting activities. This one can have a length from 7 m to 8 m. Also, oil palm fronds can constitute a 70% of the total amount of lignocellulosic residues generated in the oil palm industry. The oil palm frond is the set composed of the rachis, petiole and leaves. The petiole is the transition between the stem (rachis) and the leaves. The rachis is the stem that supports all the leaves of the oil palm tree. In Cardona Alzate et al. [66], a techno-economic analysis for an OPR biorefinery is presented for the Colombian case. The first biorefinery involves ethanol, lactic acid, xylitol and biogas production. These compounds are biochemical products obtained from fermentation and anaerobic digestion processes. The second biorefinery considers ethanol, lactic acid, n-pentane, methanol and ethyl levulinate as final products. Figure 11 shows the flow chart of biorefineries. Table 9 shows the mass balance for the biorefineries. The mass flow rate considered is 250 tons/day of OPR.
Mass balance for the OPR biorefineries as reported in Cardona Alzate et al. [66]
Product | Biorefinery I | Biorefinery II | ||
---|---|---|---|---|
Flow [kg/h] | Yield [kg/kg*] | Flow [kg/h] | Yield [kg/kg*] | |
Ethanol | 735.48 | 0.372 | 352.72 | 0.226 |
Lactic acid | 1,407 | 0.675 | 80.02 | 0.064 |
Xylitol | 1,005.34 | 0.229 | - | - |
Biogas | 2,066.21 | 1.044 | - | - |
Ethyl levulinate | 307.98 | 0.246 | ||
n-Pentane | 347.68 | 0.079 | ||
Methanol | 28.18 | 0.014 | ||
Total | 5,214.04 | 0.5 | 1,116.08 | 0.108 |
Yields expressed as mass flow of product per allocated mass flow of raw material
As a result of the analysis, a positive NPV was demonstrated with a payout period of 4.07 and 4.55 years as well as profitability indexes of 1.50 and 1.43 for biorefineries I and II, respectively. The internal rate of return for both cases was close to 50%.
OPR biorefinery proposed in Cardona Alzate et al. [66]
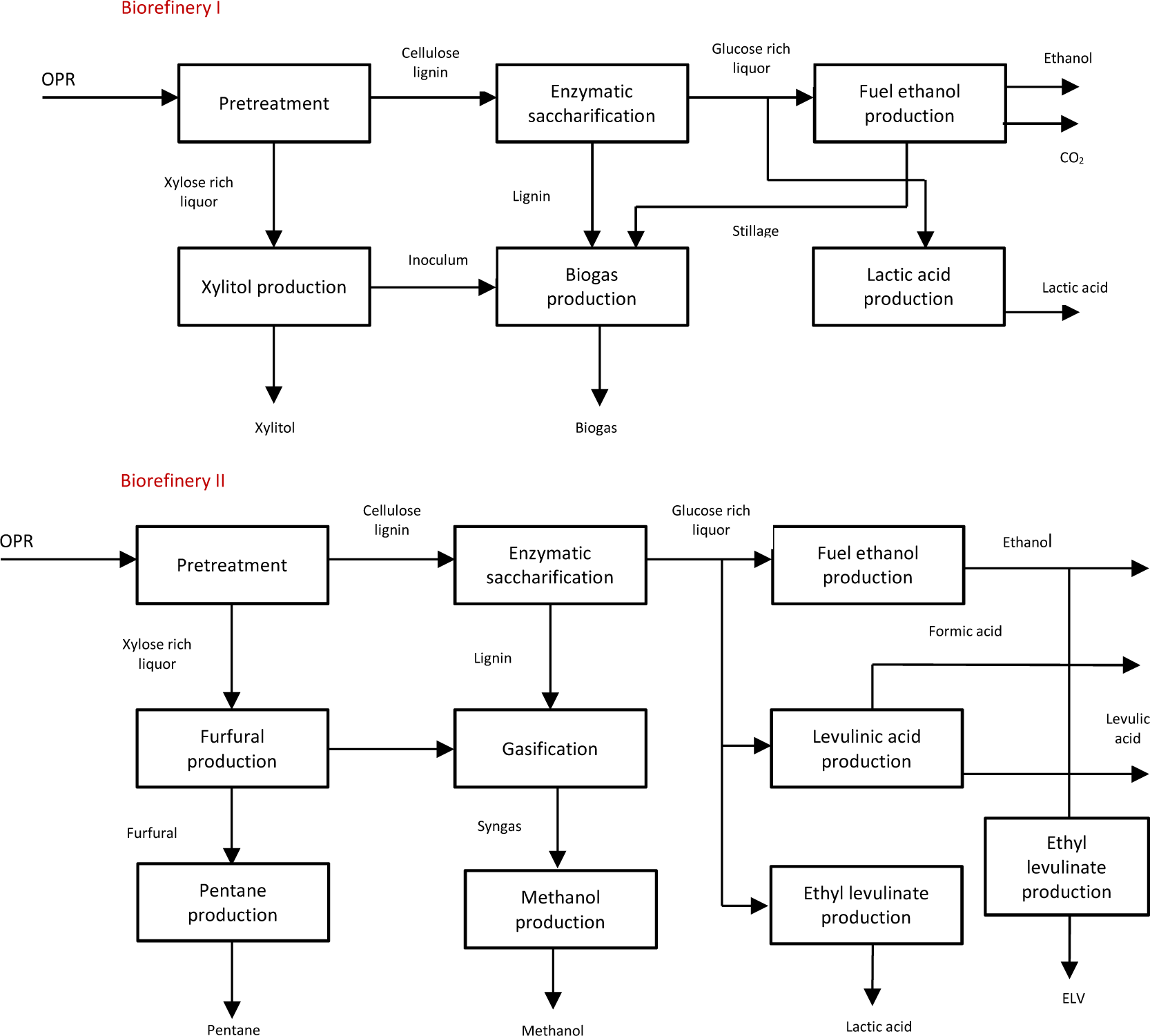
The two biorefineries analysed in this paper compare different product configurations from the same raw material. The analysis is carried out to implement catalytic processes, both homogeneous and heterogeneous. The effect of the catalytic processes implemented in the biorefinery design is mainly reflected in the total raw material and utilities costs. The implementation of catalytic processes into biorefineries increases the number of products that can be obtained from lignocellulosic biomass. Therefore, the inclusion of these types of processes could supply products to different production chains. An advantage of the catalytic processes is their facility to be implemented in any part of a biorefinery because these can be used to upgrade the main biomass components or convert the products obtained from biochemical and thermochemical pathways to other added- value products.
In Moncada et al. [40], a techno-economic analysis for a sugarcane biorefinery is presented for the Colombian case. Two scenarios for different conversion pathways for sugar, fuel ethanol, PHB, anthocyanins and electricity production are shown. The first scenario considered all the products and the second just the Colombian base case producing sugar, fuel ethanol and electricity. A simulation procedure was used in order to evaluate biorefinery schemes for all the scenarios, using Aspen Plus software that included mass balances, energy calculations and economic evaluation for each process configuration. Table 10 shows the mass balance for the scenario that involves all products.
Mass balance for the sugar cane biorefinery reported in Moncada et al. [40]
Products | ||
---|---|---|
Product | Flow | Yield |
Sugar | 240.72 tons/day | 0.05 ton sugar/ton cane |
Fuel ethanol | 193,526 tons/day | 40.32 L ethanol/ton cane |
Anthocyanin | 3.93 tons/day | 0.82 kg anthocyanin/ton cane |
PHB | 44.64 tons/day | 9.30 kg PHB/ton cane |
Electricity | 51.23 MW | 922.16 MJ/ton cane |
The results showed that the configuration with the best economic, environmental and social performance is the one that considers fuel ethanol and PHB production from combined cane bagasse and molasses (see Figure 12).
One important result to discuss in this work is that a very high profit margin is reached when all the products in an integrated biorefinery are considered (scenario 2 32.94%, see Figure 12). Another important social aspect to consider is the land requirements to ensure the needed feedstock for production. In the case of Colombia, the average crop yield per hectare is 93 tons (but in the main producing regions, it is about 120-140). Therefore, in this project a high scale is necessary and the sugarcane needed to cover the biorefinery capacity is 1,725,000 tons/year, with a land area requirement of 18,839 hectares. This land requirement represents the 8.6% of the total planted area reported for 2010 [67]. It ensures greater capacity of sugar production to keep food security, larger ethanol production for the oxygenation programme, acceptable GHG emissions, low stillage effluent and positive social aspects through job generation. PHB production would open new markets to consider second-generation feedstocks and anthocyanin production would innovate in the national market. The term sequence can be easily used for analysing other combinations of technologies, feedstocks distribution and inclusion of other products.
Scenario 2 (ethanol, PHB, sugar, energy and anthocyanins as products) for sugarcane biorefinery proposed in Moncada et al. [40]
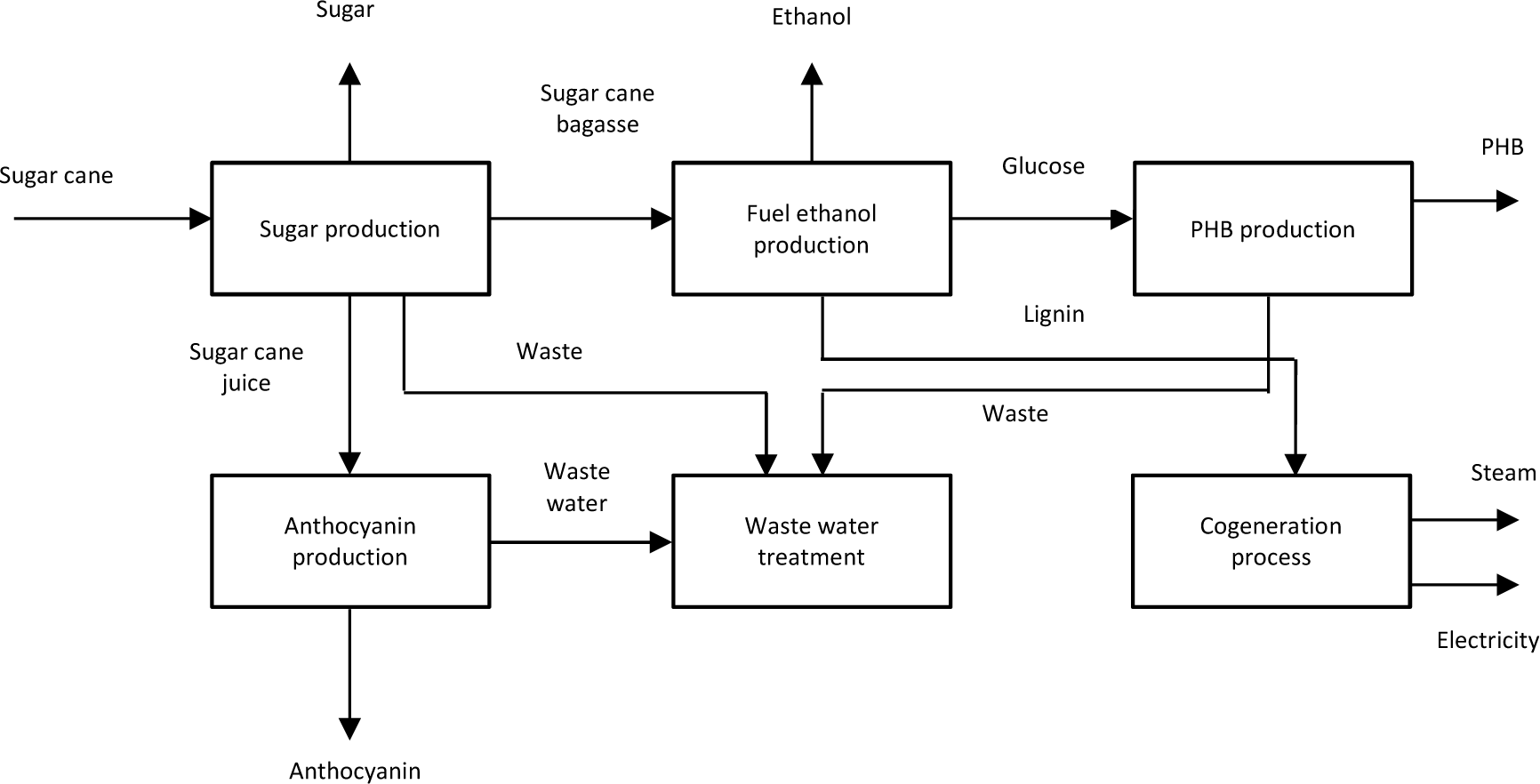
One very important topic to discuss in the sugarcane biorefinery above mentioned is the status quo that characterizes the crude-oil refineries and the sugar cane sectors. In many refineries the flexibility for a quick introduction of new products line with new equipment or revamping is really low. The same existing sugarcane factories are also very conservative and the addition of new products or conversion lines is practically impossible, even if it is a really new and lucrative business. What was learned after these statements is the importance of flexibility, especially for very big biorefineries is to be prepared for changes in the market and then in technologies or products needed to continue with the same profitability.
Beta Renewables’ commercial-scale production at the Crescentino plant in Italy is one of the most important biomass projects in the world. Beta Renewables uses lignocellulosic biomass technology called Proesa. Giant reed, agricultural and wood residues are used as raw materials. The Italy plant was designed to produce biofuels from wheat straw and corn straw in the summer, rice straw in the winter and in between winter and the summer, eucalyptus. As the web page informs [68] PROESA™ combines an enzymatic pretreatment process with fermentation. This process has a shorter residence time than other enzymatic hydrolysis technologies. It is acid and alkali-free and has minimal byproducts. The technology is protected by 26 patent family applications, 14 of which are public.
The design promised biofuel sas ethanol, bio-jet, butanol and biochemicals as fatty alcohols 1,4 butanediol, farnasene, acrylic acid, succinic acid and others. Additionally, lignin derivatives were considered as phenols, xylene, terephthalic acid. However, in 2017 it was reported that the Beta Renewables plant in Crescentino, Italy with costs of about 250 million euros, has been closed. The biorefinery had a capacity to produce 40,000 tons of bioethanol per year. This biorefinery of ethanol was the first on a commercial scale in the world. The Beta Renewables biorefinery was established at the end of 2011 as a joint venture between the subsidiary of M & G, Biochemtex and the Findo from Texas Pacific Group. Novozymes acquired 10% of the shares of Beta Renewables in 2012 [69]. In this case, logistic and some design failures can be addressed as the reasons for the operation and financing problems of this biorefinery.
Raizen is a biorefinery located in Piracicaba – SP, Brazil.
The USD 100 million plant is located adjacent to a sugar cane mill and will produce 40 million litres of cellulosic ethanol a year from sugarcane bagasse and straw. The plant construction was completed on schedule, and production of cellulosic ethanol began in late 2014. Initially, the project considered the use of bagasse as the feedstock and the return of lignin residue to the on-site boiler. The project involves Iogen technology (pretreatment, enzymatic hydrolysis and fermentation) and clearly uses the advantages of integration with a sugarcane mill [70].
The company POET-DSM Advanced Biofuels raised in 2014 is a production plant, in Emmestsburg, Iowa, United States called Project Liberty. POET is one of the largest producers of ethanol in the world. Moreover, this company is considered as a pioneer in renewable fuels. On the other hand, DSM is a recognized company in the expansion of industrial and biotechnological operations. Also, it is a leader in conversion technologies (yeast and enzymes) for cellulosic biomass to ethanol. POET-DSM is based on the strengths of both companies for the purpose of producing cellulosic bioethanol [71]. Project Liberty is now running pretreatment at 80% uptime [72]. Project Liberty, as it has been named, will process 770 tons of corn cobs, leaves, husk and some stalk daily to produce 20 million gallons of cellulosic ethanol per year, later ramping up to 25 million gallons per year. Project Liberty will be the flagship plant in POET-DSM Advanced Biofuels’ plan to license this technology to companies across the US and around the world. The company ‘announced the construction of an on-site enzyme manufacturing facility’ to directly pipe DSM enzymes into the process and ‘ramped up biomass purchasing’ in anticipation of increased production levels in 2018 [73]. Additionally, Project Liberty produces biogas and combined heat and energy [74].
Abengoa Bioenergy Biomass of Kansas (ABBK) is a company of Abengoa Bioenergy that operated a biomass-to-ethanol biorefinery located in Hugoton, Kansas, USA. This commercial scale biorefinery facility was implemented to produce 25 million-gallon/year of ethanol from nearly 350,000 tons of biomass annually and the residues to be combusted along with 300 tons/day of dry biomass material (feedstock) to produce 18-21 megawatts of electricity [75].
However, this project as one of the biggest in the world was shut down after one year of launch. The so called biorefinery, built by a Spanish company with financing from the US Department of Energy never lived up to its billing. Construction of Abengoa’s bio-refinery finished in mid-August 2014. In late November 2015, Abengoa SA, the parent company of Abengoa Bioenergy, filed for creditor protection in Spain, prompting fears it might default on nearly USD 10 billion of debt. Then the company shut down the Hugoton plant USA. Abengoa received a USD 132.4 million loan guarantee and a USD 97 million grant through the Department of Energy to support construction of the Hugoton facility. According to the Department of Energy’s website, Abengoa fully paid back its loan guarantee in March.
This is a very good case for further analysis. The financial aspects of biorefineries are still very influenced by the governments and policies. This is a very dangerous support that in this case demonstrates that the sustainability of the projects at every scale should be considered rigorously and the risks accounted before any nice project will be developed.
Biomass gasification has been widely researched since the Second World War to use hardwood as fuel for vehicles. This technology provides a relative low-cost energy production due to the amount of fuel gas that can be obtained. On the other hand, many types of gasifiers and designs have been proposed to increase the energy efficiency of the process. Solids flow, gases flow as well as the heat and mass transfer issues are considered the biomass gasification due to the feedstock properties. The main product that is obtained from the biomass gasification is a gas mixture called syngas (mainly hydrogen and carbon monoxide), which can be used as chemical platform and energy source.
Biomass gasification has not been implemented at industrial level widely. Only coal gasification has been applied to produce syngas as chemical platform. Nevertheless, co-gasification has emerged as alternative for using lignocellulosic feedstocks at industrial level in this process. Actually low-scale applications related with the heat and power production has been implemented in the world [76]. Figure 13 present the flow diagram for the gasification process.
Gasification process

Table 11 shows some developers or projects in which different raw materials are used to produce energy. Most of these projects or plants are considered as biorefineries due to the production of syngas as biofuel, electricity from syngas and in some cases the use of syngas as platform to obtain different chemicals.
Gasification plants [74]
Developer/Project | Site | Feed | Year | Capacity [MWth] | Status |
---|---|---|---|---|---|
Bioliq | Germany | PO + Char | 2017 | 5 feed | In operation |
Gobigas | Sweden | LC biomass | 2013 | 200 SNG | In operation |
GTI | USA | LC biomass | 2009 | 2 BTL | In operation |
Pyrolysis is one of the most promising thermal conversion technologies for biomass. It can decompose biomass into solid bio-char, liquid bio-oil, and combustible gas to meet different energy needs approaching the biorefinery concept. Pyrolysis processes are carried out in the absence of oxygen, at atmospheric pressure, and at temperatures ranging from 300 to 600 °C. Biochar is the main product of the traditional slow pyrolysis process, in which the biomass (usually wood) is heated slowly to temperatures between 300 and 400 °C. Fast pyrolysis, on the other hand, involves very high heating rates to temperatures around 500 °C followed by rapid cooling and condensation of the vapours produced. This yields a maximum quantity of dark-brown mobile liquid with a heating value roughly equal to that of wood, which is approximately half the heating value of fossil fuel oil [77]. Figure 14 presents a flow diagram for the process of pyrolysis.
Pyrolysis to bio-oils

Table 12 shows some companies in which different raw materials are used to produce energy and bio-oils.
Pyrolysis plants [75]
Company | Site | Feed | Year | Capacity [ML/year] | Status |
---|---|---|---|---|---|
Empyro (BTG) | Netherlands | Wood residues | 2015 | 20 | In operation |
Ensyn | Canada | Wood residues | 2006 | 20 | In operation |
Fortum | Finland | Wood residues | 2014 | 50 | In operation |
It is expected an increased growth in biorefinery projects at different scales during the next years. However, it can be concluded that the maturity of biorefineries is still very low, even when very high developed and mature technologies are applied for ethanol or electricity production as the starting point for these biorefineries operation. There should be one equilibrium between the energy and product driven biorefineries. Here the extremes are not welcome. The energy is very important for the world development but the materials and chemicals also belong to a high market demand. So, the mid-point is the best approach and all the biorefineries must include in the design a multiproduct portfolio with simultaneous production of energy, materials, chemicals and if possible, food and feed.
When crude-oil refineries are compared to biorefineries in terms of evolution and design strategies, the tendency is to repeat most of the errors from the past due to the non- inclusion of very important aspects: an integral use of raw materials, residues valorisation during and not after the design and operation, logistics analysis as well as financial stability. Most efforts are needed in the use of the modern design strategies to avoid problems in any biorefinery. A very short proposal was considered in this article. Lastly, it just means that the biorefineries should be designed from the beginning under sustainability context.
The authors express their gratitude to the Universidad Nacional de Colombia ‒ Sede Manizales and Instituto de Biotecnologia y Agroindustria for use of the facilities in which this research was conducted. The authors express their acknowledgments to the Project entitled “Biorefinería para el tratamiento de residuos de cítricos – Biorefinery of Citrus Processing Waste (CPW)” from ERANET LAC 2017 and Departamento Administrativo de Ciencia, Tecnologia e Innovacion (COLCIENCIAS ‒ ERANET-LAC) for financed this investigation. The authors express their gratitude to the Reconstrucción del tejido social en zonas de pos-conflicto en Colombia del proyecto 41858. This work has been partially financed by the Fondo Nacional de Financiamiento para la Ciencia, la Tecnología y la Innovación, Fondo Francisco Jose de Caldas with contract number 213-2018 and code 58960.
- The Rise of China’s Independent Refineries, 2017, https://energypolicy.columbia.edu/sites/default/files/CGEPTheRiseofChinasIndependentRefineries917.pdf
- An Optimization Model to Improve Gas Emission Mitigation in Oil Refineries, 2016,
- Impact of Entrepreneur on the Sectoral System of Innovation: Case Study of the Indian Crude Oil Refining Industry, 2016,
- Biorefineries: Adding Value to the Sustainable Utilisation of Biomass, 2009, http://www.ieabioenergy.com/publications/biorefineries-adding-value-to-the-sustainable-utilisation-of-biomass/
- Strategic Biorefinery Analysis: Analysis of Biorefineries, Subcontract Report NREL/SR-510-35578, 2005, https://www.nrel.gov/docs/fy06osti/35578.pdf
- Annual Energy Outlook 2015, Office of Integrated and International Energy Analysis, 2015, https://www.eia.gov/outlooks/aeo/pdf/0383(2015).pdf
- An Efficient Scheduling Method for Crude Oil Operations in Refinery with Crude Oil Type Mixing Requirements, 2016,
Design and Development of 15,000 Barrel per Day (BPD) Capacity of Modular Crude Oil Refinery Plant ,International Journal of Engineering and Modern Technology , Vol. 4 (2),pp 1-13 , 2018
, - New Jersey, USA, 2018,
- Optimal Design of Petroleum Refinery Configuration Using a Model-Based Mixed-Integer Programming Approach with Practical Approximation, 2018,
- The Biorefinery Concept: An Integrated ApproachHoboken, New Jersey, USA, 2015,
- Biorefinery Concepts in Comparison to Petrochemical RefineriesAmsterdam, The Netherlands, 2015,
- Valorization of Organic Residues for the Production of Added Value Chemicals: A Contribution to the Bio-based Economy, 2016,
- and Pérez-Ramírez, J., Environmental and Economical Perspectives of a Glycerol Biorefinery, 2018,
- Land use Competition for Production of Food and Liquid Biofuels: An Analysis of the Arguments in the Current Debate, 2010,
Task 42 Biobased Chemicals ‒ Value Added Products from Biorefineries, A Rep. Prep. IEA Bioenergy-Task, Report ,pp 36 , 2011
, - Toward a Common Classification Approach for Biorefinery Systems, 2009,
- IEA Bioenergy: Task 42 Biorefining, 2014, http://www.ieabioenergy.com/publications/biorefineries-adding-value-to-the-sustainable-utilisation-of-biomass/
- Design Strategies for Sustainable Biorefineries, 2016,
- On the Systematic Synthesis of Sustainable Biorefineries, 2013,
- Sustainable Design of Biorefinery Processes: Existing Practices and New Methodology, 2017,
- , , Biorefineries: Desing and Analysis, 2018
- Field to Fuel: Developing Sustainable Biorefineries, 2011,
Lignin for Sustainable Bioproducts and Biofuels ,J. Biochem. Eng. Bioprocess Technol. , Vol. 1 (1),pp 25-27 , 2017
, - Key Challenges and Requirements for Sustainable and Industrialized Biorefinery Supply Chain Design and Management: A Bibliographic Analysis, 2017,
- Setting the Design Space of Biorefineries Through Sustainability Values, a Practical Approach, 2018,
- Sustainable Biorefineries, an Analysis of Practices for Incorporating Sustainability in Biorefinery Design, 2017,
- The Circular Economy and the Bioeconomy Partners in Sustainability, 2018, https://www.eea.europa.eu/publications/circular-economy-and-bioeconomy
- Integrating First, Second, and Third Generation Biorefineries: Incorporating Microalgae into the Sugarcane Biorefinery, 2014,
- Design and Analysis of a Second and Third Generation Biorefinery: The Case of Castorbean and Microalgae, 2015,
- Comparative Techno-economic Assessment and LCA of Selected Integrated Sugarcane-based Biorefineries, 2015,
- Process Modeling and Analysis of Pulp Mill-based Integrated Biorefinery with Hemicellulose Pre-extraction for Ethanol Production: A Comparative Study, 2010,
- Wood Residue (Pinus Patula Bark) as an Alternative Feedstock for Producing Ethanol and Furfural in Colombia: Experimental, Techno-economic and Environmental Assessments, 2016,
- Boca Raton, Florida, USA, 2012,
- , , Chemical Process Design and Integration, 2005
- , An Overview of Chemical Process Design Engineering, Proceedings of the Canadian Engineering Education Association Conference, 2015
- Convention on Biological Diversity, Art. 2, 1992, https://www.cbd.int/doc/legal/cbd-en.pdf
- , Design and Evaluation of Sustainable Biorefineries from Feedstock in Tropical Regions, M.Sc. Thesis, 2012
- , , Conceptual Design of Chemical Processes, 1988
- Techno-economic Analysis for a Sugarcane Biorefinery: Colombian Case, 2013,
- Process Synthesis of Biorefineries: Optimization of Biomass Conversion to Fuels and Chemicals, 2014,
- Process Synthesis and Optimization of Biorefinery Configurations, 2012,
- A Systems Platform for the Optimal Synthesis of Biomass Based Manufacturing Systems, 2010,
- Early-stage Comparative Sustainability Assessment of New Bio-based Processes, 2013,
- Optimal Planning and Site Selection for Distributed Multiproduct Biorefineries Involving Economic, Environmental and Social Objectives, 2014,
- A Shortcut Method for the Preliminary Synthesis of Process-technology Pathways: An Optimization Approach and Application for the Conceptual Design of Integrated Biorefineries, 2011,
- Optimal planning and site selection for distributed multiproduct biorefineries involving economic, environmental and social objectives, 2014,
- , , Biorefineries: Integrated Biochemical Processes for Liquid Biofuels (1st ed.), 2014
- Extractive Fermentation of Acetone and Butanol: Process Design and Economic Evaluation, 1987,
- , , Efficiency of Biomass Energy: An Exergy Approach to Biofuels, Power, and Biorefineries, 2016
- , A Screening Method for Identifying Economic Improvement Potentials in Retrofit Design, European Symposium on Computer Aided Process Engineering ‒ 11, 34th European Symposium of the Working Party on Computer Aided Process Engineering, 2001
- ScienceDirect Process Systems Engineering for Biorefineries: New Research Vistas, 2013,
- The Role of Pilot and Demonstration Plants in Technology Development and Innovation Policy, 2016,
- IEA Bioenergy: Task 42 Biorefining, 2014, http://www.ieabioenergy.com/publications/biorefineries-adding-value-to-the-sustainable-utilisation-of-biomass/
- Use of Residual Banana for Polyhydroxybutyrate (PHB) Production: Case of Study in an Integrated Biorefinery, 2014,
- Techno-economic and Environmental Assessment of an Olive Stone Based Biorefinery, 2014,
- Design and Analysis of Biorefineries Based on Raw Glycerol: Addressing the Glycerol Problem, 2012,
Applications of the Solid State Fermentation in the Valorization of Agroindustrial Residues (in Portuguese) ,França-Flash Agric. , Vol. 4 ,pp 3-4 , 1995
, - Techno-economic and Energetic Assessment of Hydrogen Production Through Gasification in the Colombian Context: Coffee Cut-Stems Case, 2017,
- Design and Analysis of Antioxidant Compounds from Andes Berry Fruits (Rubus Glaucus Benth) Using an Enhanced-fluidity Liquid Extraction Process with CO2 and Ethanol, 2012,
- , Statistical Yearbook of Fruits and Vegetables 2007-2011 and its Sowing and Harvest Calendars (in Spanish), 2007
- A Biorefinery for Efficient Processing and Utilization of Spent Pulp of Colombian Andes Berry (Rubus Glaucus Benth.): Experimental, Techno-economic and Environmental Assessment, 2017,
- Analysis of Potential Technological Schemes for the Development of Oil Palm Industry in Colombia: A Biorefinery Point of View, 2014,
, Rural Mission, a Regional Perspective: Final Report , 1998
, - Potential of the Amazonian Exotic Fruit for Biorefineries: The Theobroma Bicolor (Makambo) Case, 2016,
- Fermentation, Thermochemical and Catalytic Processes in the Transformation of Biomass Through Efficient Biorefineries, 2018,
- , Annual Report 2010-2011, Colombian Sugar Sector, Association of Sugarcane Growers of Colombia (in Spanish), 2011
- PROESATM/The Scientific Research, http://www.betarenewables.com/en/proesa/the-scientific-research
- World’s ‘First’ Commercial Second-generation Bioethanol Facility ‘Shuts Down,’ Biofuel International, 2017, https://biofuels-news.com/display_news/13070/worlds_first_commercial_secondgeneration_bioethanol_facility_shuts_down/1/
- IOGEN Corporation, 2017, http://www.iogen.ca/raizen-project/
- D0SM Bright Science, Brighter Living, 2017, https://www.dsm.com/corporate/about/business-entities/dsm-biobased-productsandservices/poet-dsm-advanced-biofuels.html
- POET, 2017, https://poet.com/pr/poet-dsm-achieves-cellulosic-biofuel-breakthrough
- Breaking the Bottleneck – POET-DSM Achieves Cellulosic Biofuel Breakthrough, BiofuelsDigest, 2017, http://www.biofuelsdigest.com/bdigest/2017/11/03/breaking-the-bottleneck-poet-dsm-achieves-cellulosic-biofuel-breakthrough/
- Biorefineries in Europe (and World), 2017, https://www.smibio.net/news/2-standard/56-3rd-smibio-workshop,-cuernavaca,-mexico.html
- , Integrated Biorefinery for Conversion of Biomass to Ethanol, Synthesis Gas, and Heat, DOE Bioenergy Technologies Office(BETO) IBR 2015 Project Peer Review, 2015
- , Oil Palm Rachis Gasification for Synthesis Gas Production Oil Palm Rachis Gasification for Synthesis Gas Production, Ph.D. Thesis, 2017
- , , The Handbook of Biomass Combustion and Co-Firing, 2008